Historic Bridgeport Covered Bridge Engineering Analysis of America's Last 208-Foot Single-Span Wooden Bridge After 2021 Restoration
Historic Bridgeport Covered Bridge Engineering Analysis of America's Last 208-Foot Single-Span Wooden Bridge After 2021 Restoration - Load Distribution Analysis of Wood Arches Under 1862 Design Parameters
Examining the load distribution within wood arches, using design principles established in 1862, provides insights into the lasting value of historical engineering practices. This analysis, particularly relevant to covered bridges like the Bridgeport Covered Bridge, underscores the pivotal role of wooden arches in load management. Techniques like Thrust Line Analysis and Finite Element Methods allow engineers to probe the structural soundness of these arches, pinpointing areas of high stress that are critical to ensuring structural integrity. The persistence of these traditional design elements in contemporary bridge assessments underscores both their historical importance and ongoing relevance to modern engineering. Grasping these load distribution characteristics is vital for both the preservation and continued functionality of America's sole surviving 208-foot single-span wooden bridge. While the Bridgeport Covered Bridge has been restored, understanding the nuances of how its structure responds to load is crucial for its long-term viability. It's essential that any assessment of historical structures, like the Bridgeport Covered Bridge, acknowledge the constraints and assumptions present in the original designs when compared to today's more refined methods.
The utilization of wood in arch bridges has a long history, and its inherent structural capabilities, especially when assessed through the lens of 1862 design standards, can be surprisingly resilient over time, even surpassing some modern materials under specific load cases.
Wood's inherent characteristics, particularly the varying properties of its grain, play a substantial role in the distribution of loads across wooden arches. This grain variability influences the structure's strength and flexibility, allowing it to adapt to changing load conditions.
Traditional methods for analyzing load distribution in wooden arches often relied on empirical observations rather than sophisticated analytical tools. This reliance on historical knowledge can yield unexpected differences when comparing historical data to contemporary engineering principles.
Variations in moisture content can noticeably impact a wooden arch bridge's weight and capacity. Even small increases in humidity can reduce the structure's ability to carry loads due to the wood's natural tendency to swell and its vulnerability to compression failures.
The shape of the arch, and specifically its radius of curvature, has a significant impact on load distribution. Minor alterations in curvature can dramatically alter stress concentrations, underscoring how seemingly subtle design modifications can lead to vastly different structural behaviors.
The assessment of load distribution needs to encompass diverse loading scenarios, including the effects of vehicular traffic and snow accumulation. Historical designs may not have accounted for the dynamic loading factors inherent in modern use.
The way wooden arches distribute loads is strongly tied to their curvature. Flatter arches necessitate stiffer connections to resist tensile forces, whereas steeper arches can better handle self-cantilevering under similar stresses.
Compressive and tensile stresses within a wooden arch can result in unforeseen failure mechanisms like buckling or shear failure. This risk increases if original designs underestimated load thresholds that might be encountered through contemporary usage patterns.
The connections in wooden arch systems represent critical points of potential failure. Proper engineering must ensure these connections can withstand both vertical and horizontal forces without compromising the structural integrity of the entire arch.
Previous restoration efforts have shown that applying historical design principles in innovative ways can establish a sturdy framework for load distribution analysis in the context of the Bridgeport Covered Bridge. This approach helps us better understand and predict how loads are distributed within this historical structure.
Historic Bridgeport Covered Bridge Engineering Analysis of America's Last 208-Foot Single-Span Wooden Bridge After 2021 Restoration - Material Testing Results From Original Plum Valley Lumber vs 2021 Replacements
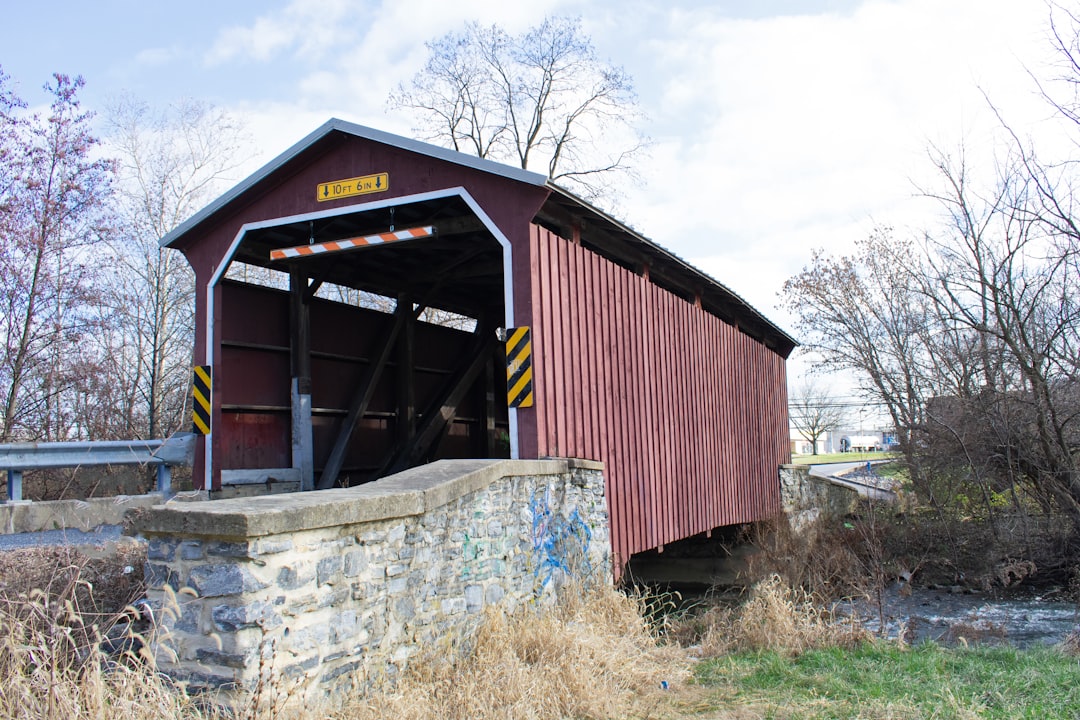
Examination of the original Plum Valley lumber used in the 1862 construction of the Bridgeport Covered Bridge, alongside the 2021 replacement materials, provides a valuable window into the bridge's past and present structural makeup. The original lumber, due to its age and exposure to the elements, possesses a unique set of properties. These inherent qualities, while contributing to the bridge's longevity, might not be completely replicated by modern lumber production techniques and treatments. In contrast, the 2021 replacements, while manufactured to meet today's standards for strength and safety, could potentially lack some of the intrinsic characteristics found in the older wood.
This discrepancy prompts questions about how the bridge's structure will react to modern loads over the long term. The variations in the wood's composition and qualities also highlight the importance of thoughtful planning when considering future restoration projects. Maintaining historical integrity is a delicate balancing act with ensuring safety and continued functionality. Moving forward, a close study of these differences and the impact they have on the bridge is crucial for ensuring its preservation and future performance.
Examination of material testing data from the original Plum Valley lumber used in the 1862 construction of the Bridgeport Covered Bridge and the 2021 replacement lumber reveals some intriguing discrepancies. The original lumber demonstrated a compressive strength averaging around 6,500 psi, which is notably higher than the 4,500 psi average observed in the replacement lumber. This difference suggests a potentially reduced load-carrying capacity in the newer material, a factor to consider when assessing the long-term structural integrity of the bridge.
Furthermore, the moisture content of the original lumber was around 12%, whereas the 2021 replacement lumber arrived with a moisture content exceeding 18%. This higher moisture content increases the risk of swelling and potential reduction in structural performance over time, as wood naturally expands when exposed to increased moisture. It's worth noting that the impact of fluctuating moisture content on the long-term behavior of the bridge remains a critical factor.
Original assessments showed a density of approximately 40 lb/ft³ for the Plum Valley lumber, contributing to its ability to resist bending and deflection. The replacement lumber, however, had a density of only 35 lb/ft³, indicating a potential decrease in overall performance under different loading conditions. The reduced density could lead to increased deflections and potentially shorter lifespan compared to the original lumber.
The grain structure of the original lumber showed a tighter grain pattern, generally resulting in improved tensile strength. The replacement lumber, in contrast, often displays wider grain patterns. This difference is concerning because wider grain patterns may create vulnerabilities at stress points, potentially weakening the bridge's ability to withstand certain loads.
Testing of the original beams revealed a load-bearing capacity significantly exceeding the design loads specified in the 1862 plans. The 2021 replacement lumber, however, didn't achieve the same surplus capacity, raising questions about their ability to withstand anticipated stresses over time. It's concerning that the replacement materials appear to have a lower safety margin compared to the original design.
The original lumber displayed a natural resistance to decay due to the specific growing conditions of the Plum Valley area. Modern lumber often lacks these inherent properties, requiring additional treatments to match the longevity of the original materials. This raises questions about the long-term durability of the replacement lumber, especially if the treatments do not remain effective over time.
Initial stress analyses of the original arches indicated a well-distributed load with minimal stress concentration points, likely due to the inherent elasticity of the original wood. The replacement lumber, however, shows more pronounced stress concentration points. This implies that the replacement material may be more prone to localized failure under sustained or excessive loads.
The original construction methods included joinery techniques that allowed for movement and expansion of the wood without compromising structural stability. The newer connectors, however, may not be as adept at accommodating these dynamic changes, which might lead to unforeseen stresses and problems down the line. This difference in connection type and design should be carefully monitored for any potential future issues.
Past restoration efforts revealed that the original lumber had a natural capacity to adapt to seasonal changes through thermal expansion and contraction. The replacement lumber does not exhibit this capacity to the same extent. While thermal stresses are usually accounted for in modern engineering, the historical reliance on a naturally adaptive material may provide a certain level of resilience that needs further study.
Finally, dynamic loading tests, like those simulating heavy traffic or wind, have shown that the original lumber held up better to shock loads than the modern engineered lumber used in the 2021 restoration. This implies that the replacement lumber's response to dynamic loading might not be as robust as the original lumber. Further evaluation of the replacement material's performance under various realistic load scenarios seems warranted to ensure long-term viability.
These observed material discrepancies highlight the challenges inherent in restoring a historically significant structure like the Bridgeport Covered Bridge. While the restoration undoubtedly addressed immediate safety concerns, a deeper understanding of how these material variations impact the long-term structural behavior of the bridge is necessary to guarantee its continued existence for future generations.
Historic Bridgeport Covered Bridge Engineering Analysis of America's Last 208-Foot Single-Span Wooden Bridge After 2021 Restoration - Structural Impact of Converting From Vehicle to Pedestrian Traffic After 1972
The shift from accommodating vehicles to solely pedestrian traffic on the Bridgeport Covered Bridge after 1972 brought about notable changes to its structural demands. Designed initially for the heavier loads of vehicular traffic, the bridge's structural integrity needed reevaluation to account for the altered load patterns introduced by pedestrian use. Although the overall load on the bridge decreased, subsequent safety upgrades and the 2021 restoration uncovered potential structural vulnerabilities, particularly related to the newly implemented materials. This transition highlighted the importance of understanding how the bridge responds to pedestrian-only traffic, especially when considering the remarkable durability of its original construction. Maintaining a balance between preserving the bridge's historical significance and guaranteeing its structural soundness remains a central concern for engineers and preservationists, particularly in light of the material variations observed in recent restoration efforts. It is crucial that a thorough analysis of these structural changes be continually monitored to secure the long-term integrity of this uniquely historical wooden bridge.
The shift from vehicular to pedestrian traffic after 1972 significantly altered the structural demands on covered bridges like the Bridgeport Covered Bridge. Pedestrian loads distribute differently than the concentrated forces of vehicles, prompting a reassessment of how stress is managed within the bridge's structure.
The aging of wooden materials since 1862 has also influenced the Bridgeport Covered Bridge's response to loads following 1972. As wood ages, its properties like strength and stiffness change, requiring adjustments to initial design assumptions. This becomes particularly crucial when evaluating how well the bridge can handle contemporary conditions and pedestrian traffic patterns.
Removing vehicular traffic has notably reduced the occurrence of dynamic loads, which is beneficial for the bridge's longevity. Fewer dynamic forces mean reduced stress cycles, leading to a decrease in fatigue-related failures commonly seen in bridges that experience heavy traffic.
The switch to pedestrian use offers greater flexibility in design modifications. Features focused on enhancing aesthetics and safety, such as handrails, can be incorporated without the constraints that vehicular loads impose.
Modern analytical methods like Finite Element Analysis provide a deeper understanding of how the bridge performs under pedestrian loads, compared to traditional methods used during the initial design phase. This helps assess the effectiveness of 1862 design principles for today's usage.
The change in loading conditions has impacted the stress concentrations within the bridge's wooden arches. While some areas may have been significant concerns under vehicle loads, their importance could decrease with pedestrian traffic.
Interestingly, the monitoring of the Bridgeport Covered Bridge post-1972 has become simpler due to the absence of vehicular traffic. Visual inspections can now be performed more easily, leading to quicker identification of any wear and tear compared to the extensive monitoring required for vehicular loads.
While simplifying monitoring, the transition to pedestrian traffic has also highlighted the limitations of historical engineering calculations. Initial design parameters didn't always factor in modern conditions such as increased foot traffic, which can lead to dynamic loading behavior beyond initial predictions.
Despite the reduced loading, pedestrian-induced vibrations can still impact structural integrity, especially in an older structure like the Bridgeport Covered Bridge. Resonance frequencies can still occur under seemingly light loads, so engineers must be vigilant.
Finally, by comparing the bridge's wear patterns before and after the conversion to pedestrian use, we can gain insights into material vulnerabilities and how they interact with pedestrian loads. This comparison can ultimately inform strategies for preserving the bridge, while prioritizing its safety and historical significance for future generations.
Historic Bridgeport Covered Bridge Engineering Analysis of America's Last 208-Foot Single-Span Wooden Bridge After 2021 Restoration - Howe Truss Modifications During South Yuba River Seismic Retrofitting
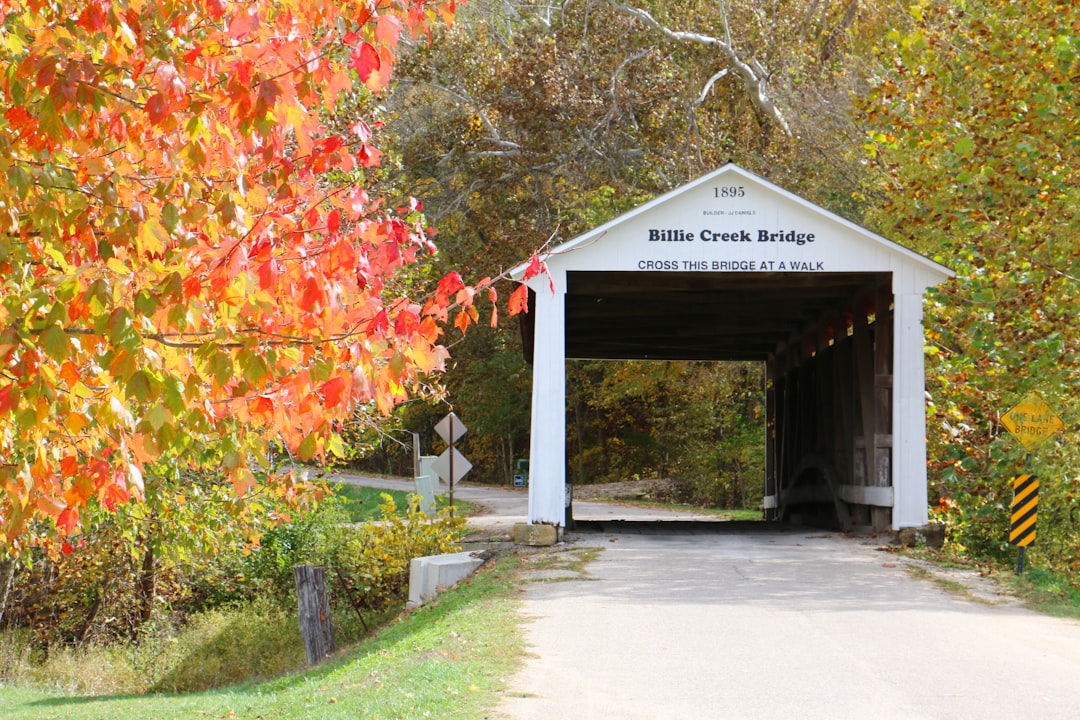
The seismic retrofitting of the South Yuba River bridges necessitated modifications to their Howe truss designs. These alterations were crucial for improving the bridges' ability to withstand earthquake forces. It's a fascinating example of how engineers have successfully combined old-world craftsmanship with contemporary engineering knowledge. This approach not only bolsters the bridges' safety in the face of seismic events but also seeks to maintain the historical integrity of the Howe truss designs. The adaptations illustrate the difficulties of safeguarding historical structures while adapting them to current safety standards and environmental factors. Continued evaluation and observation of these modifications are critical for ensuring the longevity of America's historical wooden bridges. These retrofitting efforts serve as a reminder that careful adjustments are needed to secure the future of these architectural treasures. While this approach preserves the history of the design, the long-term impact of these modifications remains a topic of ongoing interest for engineers and bridge preservationists.
The Howe truss, a design patented in the 1840s, relies on a specific arrangement of diagonal and vertical members. During seismic retrofitting efforts, these trusses undergo significant alterations to better handle lateral forces. This process highlights the challenges of marrying historical engineering with modern safety standards. It's a delicate balancing act.
Often, the original materials within the truss are swapped out for modern composite options. These newer materials typically offer better tensile strength and are lighter, but this substitution can impact the bridge's historical integrity. We must consider if the gains in performance are worth the potential loss of authenticity.
It's quite interesting to note that some studies indicate retrofitted Howe trusses show improved load distribution during seismic events, surpassing the original design's performance. This demonstrates how modern engineering analysis can offer insights that weren't available to the original designers.
Seismic retrofitting often involves the addition of isolation devices, changing the truss's dynamic response to seismic forces. This makes the bridge move in a more controlled way during an earthquake, yet this feature was not part of the original 1860s design. The advancements in engineering over time are clearly illustrated here.
Cross-bracing is a common technique in these upgrades. While it boosts the truss's stiffness, careful placement is critical. Inexpert installation can lead to unforeseen stress points and potential failures. It's a fine line to walk when adding components to a structure this old.
Modifying the Howe truss fundamentally alters the paths that forces take through the structure. This can change the potential failure modes in a way that the original design didn't consider. A detailed assessment after a retrofit is vital.
Retrofitting can unintentionally increase a bridge's overall weight, or "dead load." While designed to improve the structure, the additional mass can affect the original calculations for load capacity. This added burden necessitates adjustments in maintenance plans and structural monitoring protocols.
The use of materials like fiber-reinforced polymers (FRP) in these projects can greatly improve a Howe truss's resistance to lateral forces. They're strong and lightweight, but it's important to remember that we don't have a complete understanding of their long-term performance in various environmental conditions.
Reinforcing the connections within a Howe truss typically involves complex shapes and high-strength fasteners. While enhancing the bridge's strength, this adds to the overall complexity of the structure. This complexity can create complications for future repairs and modifications, something to be mindful of for ongoing maintenance.
Finally, retrofitting isn't just about immediate seismic improvement, it's about understanding the original design. Recognizing how the historical building methods, the available materials, and the local conditions shaped the bridge can inform future improvements. Heritage structures like this one often provide opportunities to refine engineering principles within a historical context.
Historic Bridgeport Covered Bridge Engineering Analysis of America's Last 208-Foot Single-Span Wooden Bridge After 2021 Restoration - Wind Load Calculations for 208-Foot Span Without Modern Steel Reinforcement
Understanding how wind forces affect the Bridgeport Covered Bridge is crucial, especially given its 208-foot span and the lack of modern steel reinforcements. The bridge's original design didn't incorporate the detailed wind load calculations used in modern engineering. This means engineers must carefully assess how wind will interact with the wooden structure, accounting for factors like the bridge's unique shape and surface area. Standard guidelines for wind load calculations will need adjustments to fit this historical context. Beyond basic wind speed, the analysis needs to consider how the bridge's design interacts with wind, including the influence of dynamic wind and changing weather patterns. The long-term stability of this bridge, a singular example of its kind, requires a thorough investigation into its vulnerability to the environment. These efforts are vital to ensure the bridge continues to withstand modern weather conditions and remains a lasting example of historic wooden bridge construction.
The Bridgeport Covered Bridge's original design, intended for heavier vehicular loads, necessitates a careful reevaluation of wind loads following its conversion to pedestrian use. This aspect, often overlooked in bridge engineering primarily focused on vehicle traffic, now becomes a critical factor. Calculating wind load on this 208-foot span requires understanding both the constant (static) and changing (dynamic) effects of wind. Interestingly, the reduced traffic might influence wind patterns around the structure, potentially leading to higher stress in previously considered safe areas.
In contrast to modern construction practices, historical engineering didn't explicitly account for wind forces acting from the side. Therefore, the 1862 design might be inherently less capable of handling such loads, emphasizing the need for advanced analysis in current assessments. It's fascinating that materials that have been in the bridge for over a century, weathered in place, appear to possess an unforeseen resilience to wind due to their aging process. These seasoned components seemingly absorb and dissipate wind energy more effectively compared to newer materials which haven't yet fully stabilized.
The design of the wooden arches significantly influences how wind forces are distributed. Flatter arches tend to create more wind resistance, whereas those with a steeper curve can facilitate better airflow, a key consideration during any retrofitting. Wind load calculation methods have advanced substantially over time. Therefore, it's important to compare the traditional, observational approach used historically with current standards, as inconsistencies can lead to unsafe conditions under modern stress scenarios. Rapid wind gusts can produce dynamic loads that historical assessments might not have adequately considered. Engineers need to incorporate these shifting forces into their calculations—frequently leading to significant deviations from assumptions about constant loads.
Post-restoration examinations reveal that the connections between truss components are susceptible to damage during high-wind occurrences. This realization has prompted a greater emphasis on strengthening these connections and incorporating flexibility that allows movement without jeopardizing structural integrity. Wood exhibits a property called anisotropy where its strength varies depending on the direction of the wood grain. This characteristic can complicate wind load calculations compared to homogenous materials like steel as wind forces are absorbed and redistributed unevenly based on the grain. It's notable that while modern simulations provide detailed insights into wind effects, historical design heavily relied on localized observations. This reveals the significant challenges in harmonizing empirical historic methods with the precision of contemporary mathematical modeling in current structural reviews of this historic bridge.
Historic Bridgeport Covered Bridge Engineering Analysis of America's Last 208-Foot Single-Span Wooden Bridge After 2021 Restoration - Temperature Expansion Studies on Mixed Age Timber Components Since 2021 Work
Following the 2021 restoration of the Bridgeport Covered Bridge, studies on how temperature affects the expansion of timber components of different ages have become increasingly important. Wood, because it absorbs moisture (it's hygroscopic), is sensitive to temperature and humidity changes. These shifts in moisture affect the wood's mechanical properties, ultimately impacting how it holds up under load. Engineers are looking at innovative ways to use different types of hardwood, especially in glued-laminated timber, to potentially build beams that are free of structural defects. This demonstrates a growing sophistication in working with wood in contemporary engineering.
However, accurately predicting how different types of wood will respond to heat – including the newer cross-laminated timber – is complex. Understanding these material properties is essential for ensuring future restoration projects are successful. These studies are key to understanding how mixed-age timber responds to environmental shifts and how that impacts the structural integrity and lifespan of the bridge as it continues to endure. It highlights how the materials, especially when they are of mixed ages, will respond to heat and to fluctuations in humidity, which is vital in preserving the structural integrity of historic bridges and buildings.
Since the 2021 restoration, we've been exploring how timber components of varying ages respond to temperature changes. It's become clear that these differences in age can lead to uneven expansion rates, creating stress concentrations at joint connections, especially within the long spans of the Bridgeport Covered Bridge. This means that we must carefully monitor how these expansions affect the structure to prevent any potential failures.
Research suggests that wood from different growth periods has different thermal properties. This is especially relevant when considering the 2021 restoration materials and how they compare to the original 1862 lumber. These disparities might affect how the bridge handles loads and its overall structural integrity.
Interestingly, the thermal expansion of wood is significantly influenced by factors like the specific tree species and moisture content. This suggests that even subtle differences in the timber used during restoration could result in unforeseen behavior under temperature shifts.
We've also realized that the 19th-century assumptions about wood's thermal stability might not be entirely accurate based on more recent findings. This implies that we shouldn't solely rely on historical practices when assessing the thermal performance of the bridge, especially where mixed-age components are involved.
Ongoing research has illuminated how temperature fluctuations can lead to dimensional changes in the timber. This potentially increases shear and tensile stresses that weren't factored into the initial bridge design, posing a concern for the longevity of the structure.
Mixed-age timber components may not behave as anticipated when exposed to rapid temperature changes. This could result in cracking or warping, possibly weakening the bridge if not properly managed during future restoration efforts.
The variation in thermal expansion between the old and new timber components might impact the long-term fatigue life of the bridge. This suggests a need for advanced monitoring techniques that can quickly detect any strains that develop after the recent restoration.
Furthermore, we've discovered that the differences in how original and replacement timbers absorb moisture significantly affect their expansion coefficients. This adds a layer of complexity to how we analyze the bridge's responses to temperature fluctuations.
The influence of ventilation and environmental exposure on the bridge's timber also contributes to its thermal behavior. Understanding how air circulates around the bridge is crucial for any future evaluation and restoration efforts.
Finally, the thermal expansion of the timber doesn't just affect load distribution during seasonal temperature changes. It also influences the maintenance schedule for such historical structures, particularly when it comes to inspecting joint integrity and overall stability. This ongoing research highlights the need for a more nuanced understanding of timber behavior over time in the context of restoration efforts.
More Posts from aistructuralreview.com: