Understanding Amorphous Solids Why Glass Defies Traditional Material Classification
Understanding Amorphous Solids Why Glass Defies Traditional Material Classification - Atomic Disorder The Random Dance of Glass Molecules
"Atomic Disorder: The Random Dance of Glass Molecules" explores the fundamental disarray within amorphous materials, particularly glass. Unlike crystalline structures with their predictable, repeating patterns, the atoms in glass engage in a chaotic dance, lacking any long-range order. This inherent randomness manifests in unique physical properties that challenge traditional classification systems, rendering typical material characterization methods inadequate. While the concept of short-range order provides a glimpse into local atomic arrangements, it falls short of fully capturing the intricate web of interactions that define the overall structure. Computational techniques like molecular dynamics simulations become essential tools for unraveling the dynamics of glass molecules, revealing how their random organization affects thermal and mechanical behavior, and influences their susceptibility to degradation in different environments. The study of glass, with its inherent atomic disorder, compels us to develop a richer understanding of the broader category of non-crystalline solids and their peculiar behaviors, highlighting the intricate interplay between disorder and material properties.
Amorphous materials like glass pose a significant challenge to our conventional understanding of materials, because they lack the long-range order that defines crystals. This absence of periodicity makes it difficult to apply traditional tools to characterize their internal structure. When we talk about "glass" in a scientific context, we specifically mean amorphous solids that undergo a glass transition, which is a unique transformation that separates them from their crystalline counterparts.
While the concept of short-range order (SRO) helps us understand the arrangement of atoms in close proximity, it doesn't offer a complete picture of the overall structure. The broken symmetry at the atomic scale introduces complications in determining proper metrics for understanding the properties of glasses. The interplay of molecules, as seen in materials like borosilicate glass, plays a crucial role in their behavior, particularly when they interact with other substances, such as water, during degradation processes.
We employ techniques like molecular dynamics simulations to understand the complexities of these materials. However, it's important to remember that "glassy solids" are just a specific subset of a larger category—noncrystalline solids—which encapsulate a wider array of materials displaying structural randomness. Examples like silica gels, with their intricate networks of interwoven structures, beautifully highlight the variety of arrangements possible in these materials.
The randomness inherent in glass creates a situation where it doesn't fit neatly into the standard categories of materials. This uniqueness extends to its thermal and mechanical properties, giving it a fascinating blend of characteristics that defy simple classification. Its structure fundamentally alters how it responds to changes in temperature or force, providing a fertile ground for further research.
Understanding Amorphous Solids Why Glass Defies Traditional Material Classification - Non Crystalline Structure Breaking Traditional Material Rules
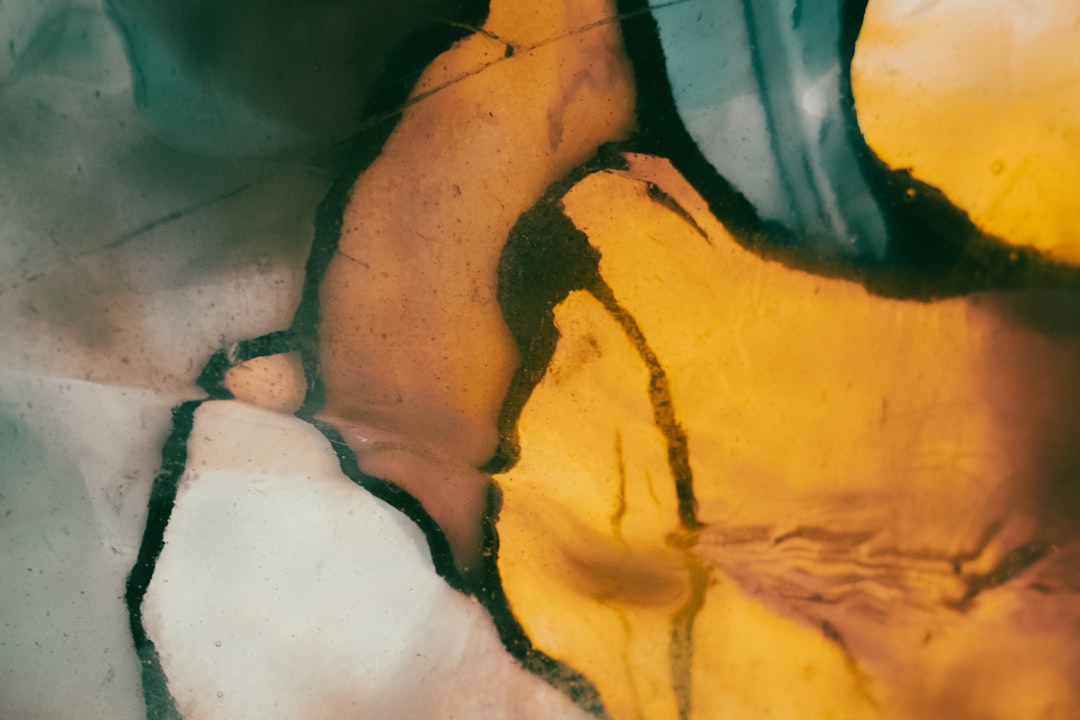
The defining characteristic of amorphous materials, including glass, is their non-crystalline structure, which fundamentally deviates from the established rules governing traditional materials. This absence of long-range atomic order presents a significant challenge to standard characterization techniques, forcing a reevaluation of how we classify and categorize solids. The distinction between glasses and other amorphous materials within this broader category becomes increasingly crucial as they exhibit unique behaviors that don't easily fit into existing frameworks.
The structural ambiguity of these non-crystalline solids makes predicting their mechanical and thermal properties challenging. Their behavior often defies simple categorization, highlighting the limitations of conventional material classifications. The ongoing study of amorphous materials, including emerging research on metallic glasses and coordination polymers, is revealing a more complex landscape of possible structures and behaviors than previously understood, pushing us to refine our understanding of the fundamental nature of these materials.
Amorphous solids, including glass, challenge traditional material science because of their non-crystalline structure. Their lack of long-range order leads to some unusual characteristics. For instance, glass displays remarkable thermal stability, resisting rapid temperature shifts better than many crystals, which is quite useful in various applications like optics and electronics.
The optical clarity of glass stems from its disordered structure, which uniquely scatters light less than crystals. This transparency is a key reason for its widespread use in lenses and fiber optics.
The mechanical behavior of amorphous materials can be different than in crystalline materials. Glass, for example, can handle a lot of stress before breaking because of the absence of the weak points known as grain boundaries, but it also has a tendency towards brittle failure.
Glass transition, a key characteristic of glasses, is not your typical phase transformation. Instead of a sudden shift, it's a gradual increase in viscosity, creating a smooth transition from solid-like to fluid-like behavior. Understanding how this gradual transition works could provide important insights for materials development.
We can control the atomic disorder in amorphous solids by carefully tuning their composition. Adding elements like sodium or alumina to the glass network affects its connectivity and therefore its overall properties. This compositional flexibility opens up a wide range of possibilities for tailored materials with specific desired properties.
The mechanical response of amorphous materials can vary drastically depending on how quickly a load is applied (strain rate sensitivity). This can be a factor in engineering design, especially when dealing with impacts or shocks.
The non-crystalline structure of amorphous solids sometimes facilitates faster atomic diffusion compared to their crystalline counterparts. This is important in processes like ion exchange, where properties of glasses can be modified in-situ.
Interestingly, amorphous solids, while seemingly isotropic on a large scale, can have anisotropic behaviors in some situations, meaning that their properties can change depending on the direction you measure them. This is contrary to what is often assumed about materials, making the behavior of these materials quite fascinating.
A key issue in materials science regarding amorphous solids is glass-forming ability. Certain materials are naturally good at forming a glass when cooled, while others require specific processing conditions. This reinforces that not all non-crystalline materials are equally able to form a glassy state.
Finally, amorphous solids can experience aging, which is a change in their properties over time, often causing them to become more brittle or alter their mechanical behavior. The implications of aging effects can influence decisions regarding the long-term use of these materials. It is a critical factor for scientists and engineers to consider.
This is just a glimpse into the unusual nature of amorphous solids, and much more remains to be discovered regarding their fundamental structure and behavior. The peculiar properties of these materials demonstrate the power of disorder, prompting us to reevaluate our traditional understanding of materials and potentially unlock innovative possibilities in design and applications.
Understanding Amorphous Solids Why Glass Defies Traditional Material Classification - Network Modifiers How Chemical Elements Shape Glass Properties
Network modifiers are crucial elements that alter the fundamental structure and properties of glass. Unlike network formers like SiO2 that create a continuous network, modifiers introduce disruptions by incorporating elements like sodium, calcium, and aluminum into the glass structure. These elements, primarily in the form of oxides, dissociate within the glass and contribute cations that influence the overall characteristics. The arrangement and concentration of these modifiers can vary depending on the glass type, with their valence order impacting the stability and overall behavior.
The ability to control the ratio of network formers and modifiers allows for the creation of glasses with customized properties. By carefully adjusting this balance, researchers can design glass tailored to specific applications. This compositional control not only alters the glass's structure but also influences other aspects like how it ages, its response to mechanical forces, and its optical characteristics. It highlights the interconnected nature of chemical composition and material performance in the intriguing world of amorphous solids.
Network modifiers, such as sodium and potassium oxides, play a crucial role in shaping the properties of glass by disrupting the inherent silica network structure. These elements introduce non-bridging oxygen atoms, altering the glass's overall stability, particularly in terms of its thermal and chemical resistance. However, this modification often comes at the cost of mechanical strength, illustrating a common trade-off in material design.
The presence of these modifiers can significantly lower both the melting temperature and viscosity of glass. This makes it easier to process and shape during manufacturing, enabling production of more complex glass forms efficiently. For instance, when we reduce viscosity, it becomes easier to make intricate glassware, or fiber optic cables. However, it's vital to balance these processing advantages against potential consequences for the glass's end-use stability.
Beyond structural alterations, network modifiers influence ion mobility within the glass. This is especially notable with sodium, which increases the glass's ionic conductivity. This property makes certain glasses highly suitable for applications involving batteries and other electrochemical devices where charge transport is essential.
The impact of network modifiers on a glass's chemical durability can be quite complex. Enhancing resistance to thermal shock can unfortunately lead to reduced chemical resistance in some cases. Soda-lime glass is a prime example of this compromise—while economical and versatile, it's prone to corrosion in alkaline environments, unlike more chemically resistant glasses like borosilicates or high-silica types.
The composition of the glass, specifically the types and proportions of network modifiers, has a profound influence on its final properties. This compositional flexibility is what makes it possible to engineer glasses for specialized applications. For instance, optical fibers or highly robust containers require very specific compositions achieved through careful control of these network modifiers.
The introduction of specific network modifiers can create unexpected anisotropic behavior in glass. Usually glass is considered isotropic, meaning its properties are the same in all directions. However, this is not always the case with network modifiers. This unexpected anisotropy has significant implications for the design of materials where directionally-dependent properties, like strength or conductivity, are desired.
The glass transition temperature, which marks the shift between solid-like and liquid-like behavior, can be heavily influenced by the presence and types of network modifiers. This aspect is critical to understand when designing or processing specific types of glass, as it determines many process parameters.
Interestingly, network modifiers can influence the crystallization behavior of glasses. In some cases, they can promote crystal formation, while in others they can inhibit it. This has profound consequences for the final product, as even slight shifts in crystal content drastically affect properties like clarity or mechanical strength.
The long-term performance of glasses is also impacted by the presence of modifiers. Some compositions can lead to accelerated aging, which can lead to changes in mechanical properties over time, particularly a tendency towards brittleness. This aging effect is especially important when choosing materials for applications where long-term stability is paramount.
Finally, network modifiers can significantly alter the optical properties of glass by changing the refractive index. This is a crucial consideration in the design of optical devices where light manipulation is fundamental, like lenses or optical fibers. Even small variations in the type or amount of modifiers can lead to large shifts in light transmission and clarity, emphasizing the importance of careful composition control.
In conclusion, understanding network modifiers is essential to appreciating the full complexity of glass as a material. The seemingly simple act of adding specific elements can dramatically alter a wide range of properties, revealing a delicate balance between desirable and undesirable traits that require thoughtful consideration for specific applications. Continued research into how network modifiers influence glass behavior is likely to unlock even more possibilities for designing glasses tailored to specific functional demands in diverse engineering fields.
Understanding Amorphous Solids Why Glass Defies Traditional Material Classification - Mechanical Behavior Understanding Glass Fracture Patterns
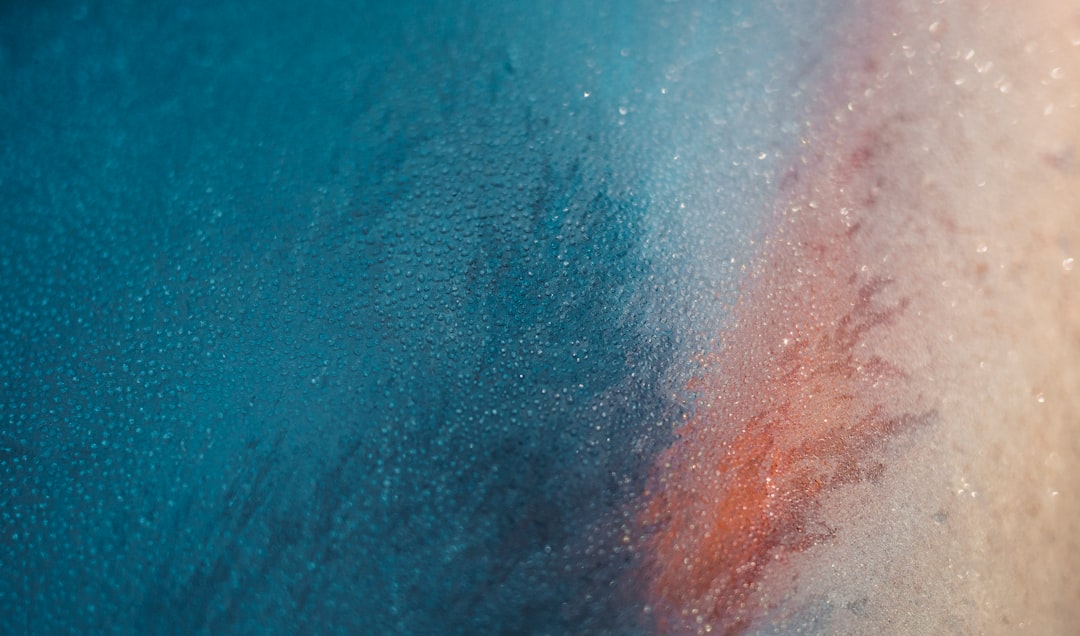
Understanding how glass responds to mechanical forces and breaks, or fractures, unveils the intricate nature of this seemingly straightforward material. A key aspect is fracture toughness, which quantifies a material's resistance to catastrophic failure when a crack is present. This property is particularly important for glass due to its tendency towards brittle failure. Examining the distinct patterns of cracks can offer valuable clues about how a piece of glass was stressed and ultimately broke. For example, chemically tempered glass often shows cracks that are linear or slightly branched, while high-strength glass might exhibit more complex, interconnected crack networks.
Further, the speed at which cracks travel during fracture reveals important characteristics of the material's internal structure, showing us how the disordered nature of glass impacts how cracks propagate. Additionally, observations suggest that tiny voids or cavities (cavitation) play a crucial role in how glasses break, pointing to a potentially common mechanism across many types of glass. By studying the surfaces left behind after a fracture, we can learn more about the conditions that caused it, gaining insights into the energy involved in the breakage process. This growing understanding of fracture patterns and mechanisms in glass can ultimately lead to improved predictions of its performance and broaden the possibilities for its use in diverse engineering applications.
The mechanical behavior of glass, particularly its propensity for brittle fracture, is intrinsically linked to its atomic structure. Understanding the patterns of fracture in glass offers important clues into the mechanisms that govern its failure. For example, the presence of hackle marks – a type of surface feature – reveals the rapid and often catastrophic nature of crack propagation, a stark contrast to the more ductile deformation observed in metals before they break. This difference emphasizes the unique mechanical nature of glass compared to more traditional materials.
Furthermore, the absence of grain boundaries in glass's structure alters how stress concentrates within the material. While we often look at a material’s overall atomic arrangement to determine mechanical properties, in the case of glass, it's often minor defects or imperfections that significantly impact fracture behavior. This means it's critical to rigorously control the manufacturing process to minimize the presence of these flaws and potentially predict the onset of fracture in glass products.
Interestingly, these so-called "critical flaws" can be more informative about the mechanical behavior of glass than the material's average structure. Understanding their locations and sizes is essential for predicting potential failure. This contrasts with many other materials, where a material's average characteristics are often a good first-order estimation of mechanical strength.
Temperature also has a significant impact. At higher temperatures, near its glass transition point, the mechanical properties of glass drastically change, becoming more ductile and even somewhat flexible. This behavior is a far cry from the brittle, easily breakable solid glass typically encountered at room temperature.
The rate at which a load is applied to a glass structure is also important. Sudden and rapid loading can trigger brittle failure, whereas slower loads sometimes permit a more complex behavior called viscoelasticity which might lead to a different fracture path.
However, the inherent fragility of glass makes it significantly less resistant to fracture than many crystalline materials. Engineers need to design glass structures that are often thicker or use reinforced frameworks to help improve their durability against the impact of unexpected forces or stresses.
The initial formation of microcracks in a piece of glass can often foreshadow macroscopic fractures. Understanding these micro-scale events early on is crucial for ensuring the safe and reliable performance of glass structures and predicting the material's lifespan.
While usually regarded as isotropic, meaning its properties are the same in all directions, glass can actually exhibit anisotropy under certain loading conditions. This signifies that the response of a glass structure depends on the orientation of the applied stress, leading to a more intricate failure behavior than initially expected.
The presence of water can significantly worsen the tendency for cracking in some glass structures. Certain glasses may experience enhanced bond weakening when exposed to moisture, leading to a noticeable increase in their susceptibility to fracture.
The study of fracture surfaces, known as fractography, is a valuable technique to probe the root cause of glass failure. This is because the fracture surface provides a historical record of the events that led to breakage. Through careful examination, we can reconstruct the stress history, the type of load that caused the break, and develop designs that minimize catastrophic failure.
Overall, glass presents a rich tapestry of fracture patterns and behaviors influenced by its inherent disordered structure, temperature, and the application of external forces. A deeper understanding of these mechanisms has implications for engineering stronger and more durable glass products and designing more resilient systems that incorporate glass components.
Understanding Amorphous Solids Why Glass Defies Traditional Material Classification - Manufacturing Methods From Sand to Transparent Sheets
The journey from sand to transparent sheets of glass involves a series of manufacturing processes that underscore the unique, non-crystalline nature of this material. The fundamental component, silica, largely sourced from sand, undergoes a transformation when heated with various additives, resulting in a molten state. This molten mixture can then be shaped into a variety of forms, with methods like the float glass process enabling the creation of large, uniformly flat sheets vital for applications relying on optical properties. However, the characteristics of the finished glass are not solely determined by the initial raw materials. Careful manipulation of the chemical composition throughout the manufacturing process significantly influences the resulting material's thermal stability, mechanical properties, and optical clarity. This intricate interplay between processing techniques and chemical composition challenges conventional classifications of glass as a solid material, highlighting its distinct behavior and expanding our understanding of its role across diverse technological and artistic fields.
Amorphous solids, often referred to as glass, represent a fascinating challenge to conventional material classifications. Their unique properties arise from a fundamental lack of long-range order at the atomic level, a characteristic that sets them apart from crystalline materials.
Creating glass from sand requires high temperatures—around 1700°C—to melt the silica (SiO2) found in sand. This high-energy process breaks down the rigid SiO2 structure, allowing it to transition into a viscous liquid that can then be shaped. The glass transition temperature, a defining feature, is where the material shifts from its typical brittle, solid state to a more fluid, malleable state. This is where it's often shaped into the various products we encounter daily.
Adding other oxides like sodium or calcium during this process profoundly alters the final properties of the glass. This compositional flexibility is key to producing glasses with specific characteristics, whether it's lowering the melting point or enhancing durability. While considered a solid, glass retains a subtle liquid-like behavior below the glass transition point. This "hidden" mobility means the glass can very slowly rearrange its atomic structure over extended periods, which can affect properties like its strength over time.
Examining how glass breaks reveals much about its internal structure. The nature of cracks can tell us about the stress a piece of glass experienced. Further, small imperfections within the glass, such as tiny voids, can act as stress concentrators, influencing how cracks develop. This sensitivity to flaws highlights the importance of tightly controlled manufacturing processes. Moreover, glass’s mechanical properties are heavily influenced by temperature, with the material becoming more pliable near the glass transition point. It's a classic example of how sensitive glass can be to its surrounding environment.
Glass's mechanical behavior can also change over time via aging. This slow alteration in the structure can lead to the glass becoming more brittle over time. Understanding this aging effect is particularly important in designing structures where glass will bear a load for a long time. The peculiar disorder within glass gives it the optical clarity that is prized. Compared to crystals, where light can scatter off of regular atomic structures, the random nature of glass allows light to pass through without significant interference.
These observations highlight how glass defies traditional material classifications. It's a solid that shows subtle liquid-like behavior, sensitive to temperature, prone to aging and changes with composition, and prone to brittle fracture. It requires researchers to think about materials in a different way, leading to insights that could be applied to other materials as well. The challenges glass poses for our understanding of solids could ultimately lead to new discoveries and materials with unique behaviors and applications.
More Posts from aistructuralreview.com: