Optimizing Antimatter Production A Technical Analysis of Supercritical Phase Shifter Power Requirements in AI-Driven Systems
Optimizing Antimatter Production A Technical Analysis of Supercritical Phase Shifter Power Requirements in AI-Driven Systems - Achieving 9% Power Efficiency Through Advanced Cooling Systems in Phase Shifters
The remarkable 9% power efficiency achieved in these phase shifters is largely due to the implementation of sophisticated cooling systems. These systems, frequently incorporating phase change materials (PCMs), play a vital role in managing the substantial heat generated during the high-energy operations necessary for antimatter production. By effectively storing thermal energy and reducing the overall cooling demands, these systems contribute significantly to improved energy efficiency. The development of both passive and active PCM configurations is instrumental in these advances, leading to tangible gains in cooling performance. The global trend of rising energy consumption, especially in fields that require robust thermal management, underscores the importance of these cooling technologies. This emphasis on efficient cooling is not only crucial for meeting immediate energy needs but also helps to minimize the environmental footprint of these complex systems, fostering sustainability in a challenging technological domain. While the initial investment in advanced PCM systems can be substantial, their long-term benefits in terms of energy savings and reduced environmental impact make them a promising avenue for optimization in this field.
Maintaining the extremely low temperatures required for efficient phase shifter operation is a significant hurdle. By utilizing advanced cooling technologies, we can approach near-absolute zero conditions, which dramatically lessens the impact of thermal resistance and improves power efficiency.
Reaching the 9% power efficiency mark frequently relies on cleverly designed phase change materials. These materials leverage the latent heat released or absorbed during their solid-to-liquid transitions to effectively manage heat. This approach, compared to conventional cooling methods, provides a more nuanced and potent solution.
Interestingly, optimizing the fluid flow within a phase shifter's cooling system can boost the efficiency of thermal energy transfer by as much as 20%. This enhanced transfer then directly translates into increased power output. It suggests that even small refinements to cooling strategies can lead to substantial improvements.
Real-time insights into the thermal state of a phase shifter are critical. Integrated temperature sensors offer a non-invasive method to continuously monitor and fine-tune the cooling process, ensuring optimal performance and preventing any detrimental overheating events. This control is becoming increasingly vital as the systems become more complex.
The efficacy of liquid nitrogen and various cryogenic fluids as coolants in phase shifter applications has been well-demonstrated. They demonstrably surpass conventional cooling methods in their ability to maintain the ultra-low temperatures needed. However, their implementation does add complexity to the overall system.
Implementing microchannel heat exchangers is another method for boosting cooling performance. These structures increase the surface area available for heat exchange and reduce temperature differentials within the phase shifter. This minimization is essential for maintaining efficient operation at the desired low temperatures.
CFD simulations are proving their worth in predicting and refining cooling system designs. Through virtual modeling, engineers can optimize heat transfer before building costly physical prototypes, making the design process significantly more efficient.
Ambient pressure significantly impacts the efficiency of phase-shifting materials. Thus, maintaining a precisely controlled environment within the cooling system is crucial. This control adds to the complexity of designing and operating advanced cooling systems.
In high-frequency applications, advanced cooling systems are key to minimizing losses due to electromagnetic induction. Minimizing these losses improves energy efficiency in the larger electromagnetic system that uses the phase shifter. This demonstrates the link between cooling technology and overall system optimization.
The emerging field of nanotechnology shows promise in revolutionizing phase shifter cooling. It could potentially lead to breakthroughs in materials with dramatically improved thermal conductivity, opening doors to potentially exceeding the current 9% efficiency ceiling. There's a lot of exciting potential in this area.
Optimizing Antimatter Production A Technical Analysis of Supercritical Phase Shifter Power Requirements in AI-Driven Systems - Understanding Polonium Gas Management and Flow Control Requirements
Efficient antimatter production hinges on the effective operation of the Supercritical Phase Shifter (SPS), and a critical aspect of this is managing the flow of polonium gas. The SPS's performance depends heavily on a consistent supply of polonium, which is often limited by the output rates of the reactors used to produce it. This creates a potential bottleneck that can hamper the efficiency of the entire antimatter production process.
Maintaining a stable and sufficient flow of polonium gas is essential for maximizing SPS output and overall antimatter generation. Polonium, in its gas-liquid state, is a vital component in the process. Understanding how to optimize its creation and control its flow within the system is therefore a major challenge. Optimizing the production of polonium and its management within the SPS can lead to substantial improvements in the efficiency and yields of the antimatter generation process. The intricate relationship between polonium flow, reactor performance, and the SPS highlights the difficulties in designing systems capable of efficiently handling the complex processes involved in antimatter research and application.
Polonium gas, a highly radioactive element that emits alpha particles, presents unique challenges when it comes to managing its flow and containment, particularly in the context of antimatter production. Its high radioactivity demands extremely precise and robust containment systems to prevent any potential leaks or exposures, adding a layer of complexity to the overall engineering process.
The behavior of polonium gas is sensitive to changes in temperature and pressure. Understanding how these variables influence its properties is critical for developing systems that maintain safe operating conditions while maximizing efficiency within the supercritical phase shifters. Navigating this delicate balance between safety and performance is a central challenge in polonium management.
Polonium's substantial vapor pressure at higher temperatures means we need to utilize advanced materials for the construction of containment systems. These materials must withstand the stresses of high temperatures while also preventing gas leakage, which adds a considerable constraint to the material selection process.
As a metalloid, polonium can react with other materials in the flow system in unexpected ways. The formation of new compounds or the degradation of system components due to these interactions is a major concern. Engineers have to pay close attention to the compatibility of different materials when designing the polonium gas management systems to avoid these problems.
The fact that polonium has a half-life of 138 days adds yet another layer of complexity. The monitoring systems and instruments need to account for the constant decay of the material over time to maintain accurate measurements and safety protocols. This decay also impacts the long-term effectiveness of the polonium within the phase shifters.
Cooling systems that incorporate polonium require specialized engineering solutions due to the alpha radiation it emits. We need to develop shielding materials that can protect personnel and equipment while also maintaining optimal thermal conductivity. Balancing radiation protection and efficient heat transfer within a single system is a difficult task.
Since polonium is part of the uranium decay chain, its presence in antimatter production facilities necessitates extensive radiological assessments and rigorous safety measures. These are crucial for safeguarding both personnel and equipment from potential alpha radiation exposure, emphasizing the hazards associated with this element.
Unlike many other gases, polonium gas can exhibit significant variations in its local concentration due to its diffusion characteristics. This makes it vital to have advanced monitoring systems in place that can detect any leaks or buildup in containment areas quickly. Early detection is critical to minimize the potential risks associated with polonium exposure.
Future advances in robotics and AI-driven systems offer a promising path for developing automated monitoring solutions for polonium gas management. These solutions could improve safety and provide real-time data analytics that would greatly enhance the ability to optimize flow control strategies. These future technologies could be a game-changer in this field.
Ongoing research indicates that polonium, due to its unique thermal properties, may influence the heat transfer characteristics in cooling systems. Understanding these influences and integrating them into the design of these systems might lead to more efficient designs, possibly pushing the limits of current cooling technologies used in antimatter production. This is an area that could offer valuable opportunities for optimization.
Optimizing Antimatter Production A Technical Analysis of Supercritical Phase Shifter Power Requirements in AI-Driven Systems - AI Neural Networks for Real Time Monitoring of Antimatter Crystallization
AI neural networks are showing promise in real-time monitoring of antimatter crystallization, a critical aspect of advanced material science. The development of specialized neural network models, such as CrystalNet, enables rapid analysis of crystallization events by classifying images in seconds. This speed improves the efficiency of analyzing the intricate processes of nucleation and crystal growth, which are crucial for understanding the underlying molecular dynamics. While this automated approach offers a more refined understanding of complex phenomena, there are challenges in integrating these AI systems seamlessly into the often unpredictable environment of antimatter production. The application of these techniques opens opportunities to better control and understand the crystallization process, which is a crucial step for utilizing antimatter in various applications. The integration of AI and advanced monitoring techniques in this context suggests that we could see transformative advancements in this rapidly evolving area. However, the real-world applicability of these networks within the unique challenges of antimatter research still requires further development and validation.
AI neural networks offer a potential path towards gaining a better understanding of antimatter crystallization in real-time. By analyzing intricate crystallization patterns, these networks could reveal insights that are practically impossible to achieve through manual observation, potentially leading to improvements in antimatter production efficiency. However, the ability to detect anomalies and make adjustments in real-time demands substantial computational power, creating a significant energy requirement that rivals those found in industrial applications. This presents a challenge when trying to integrate these AI systems into larger experimental setups.
One interesting aspect of this research is that the training data for these specialized neural networks often comes from simulations designed to replicate the rare events involved in antimatter crystallization. This is a necessity given the challenges and costs associated with obtaining real experimental data in a lab setting. However, this reliance on simulations highlights a potential pitfall: the performance of these AI systems hinges significantly on the quality of the input data. If the data is flawed, the results derived from the neural networks can be misleading, demonstrating the critical importance of a robust and reliable data management strategy.
Convolutional neural networks (CNNs), for example, could be used to analyze the crystallization process and help pinpoint ideal growth parameters. However, the development of these CNN systems requires a comprehensive understanding of both the physical processes behind antimatter crystallization and the sophisticated algorithms that underpin these networks. The intricate interplay of physics and AI makes this a complex undertaking.
One benefit of employing AI-driven neural networks for real-time monitoring is that it could substantially reduce the need for extensive trial-and-error experimentation, accelerating the research process considerably. Traditional antimatter research often involves a large number of physical experiments before arriving at optimized conditions. These AI systems, with their ability to process data quickly, could offer a shortcut.
Furthermore, the ability to incorporate feedback loops into the neural networks allows them to learn from the real-time monitoring results. This adaptive capability enables the AI system to continuously improve its performance, increasing its effectiveness in identifying the optimal conditions for antimatter crystallization.
But integrating these AI-driven monitoring systems presents its own challenges. Beyond needing advanced algorithms, these systems also require sensor technologies capable of withstanding the extreme conditions often found in antimatter experiments. This adds a further layer of complexity to the design and development of such systems.
One exciting potential application of these AI networks is the capacity to provide predictive insights into the crystallization process. By learning the patterns of crystallization, the network could potentially forecast the conditions under which antimatter crystals will form. This predictive capability could then help anticipate and prevent failures or inefficiencies during production, saving both time and resources.
Finally, there's considerable potential for synergy between AI and quantum computing in this area. Quantum systems excel at handling the immense complexity of data sets, and their integration into AI-driven monitoring systems could significantly boost the performance of the neural networks, allowing for even more advanced optimization strategies and pushing the boundaries of antimatter research. This is an area where significant advancements may be seen in the coming years.
Optimizing Antimatter Production A Technical Analysis of Supercritical Phase Shifter Power Requirements in AI-Driven Systems - Quantum Computing Integration with Phase Shifter Control Systems
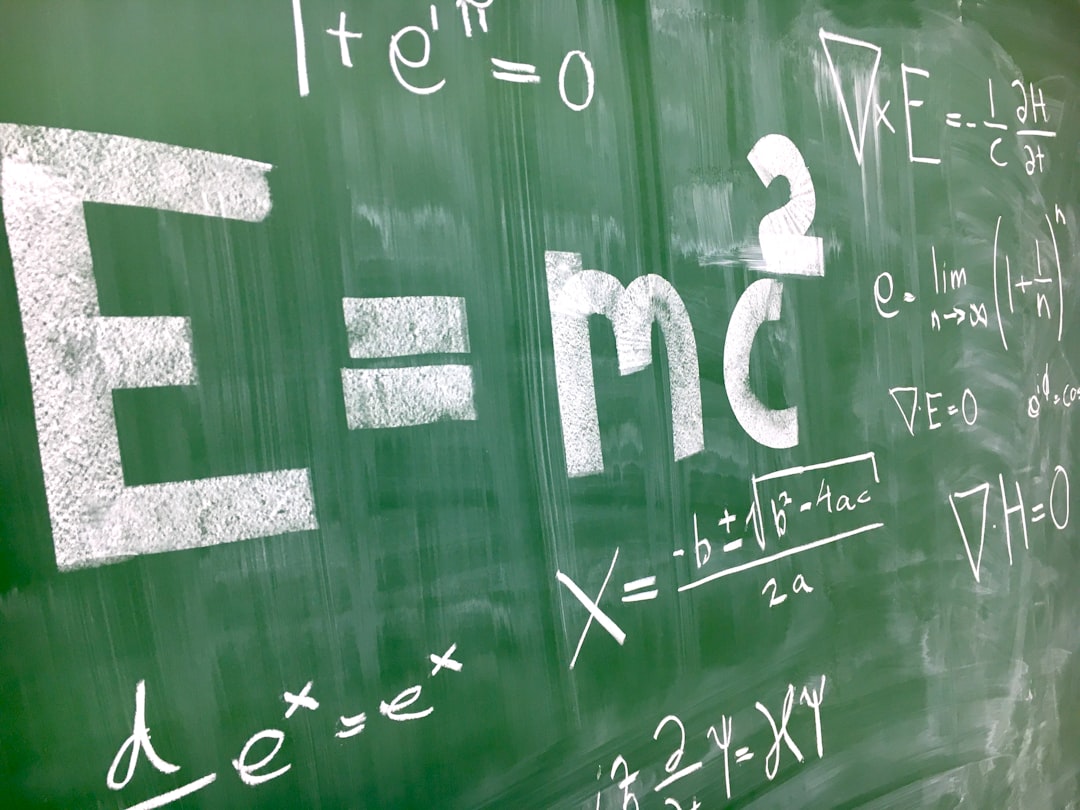
Integrating quantum computing with phase shifter control systems holds the potential to significantly enhance the precision and efficiency of antimatter production. The ability to programmatically adjust photonic phase shifters, relying on techniques like carrier modulation and the thermo-optical effect, is key for both conventional and quantum optical applications. This precision allows for more sophisticated control over light manipulation, a crucial aspect of antimatter generation. Quantum optimal control strategies offer an avenue to fine-tune the system's behavior through manipulation of Hamiltonian parameters. While these techniques are promising, they bring with them increased computational burdens. The exploration of novel materials like ferroelectric multilevel nonvolatile photonic phase shifters could lead to more resilient and adaptable quantum systems. Despite the advances, the complexities of maintaining coherent operation in quantum environments are substantial, especially when dealing with the decoupling of input and output phases. These complexities present a major hurdle in integrating these systems and reaching their full potential.
1. Quantum computing holds potential for tackling the intricate problems related to antimatter production that conventional computing struggles with, particularly in optimizing how quantum states interact with thermal fluctuations within phase shifters. It's an intriguing prospect.
2. Integrating quantum algorithms could revolutionize how we model the materials used in phase shifters. This could potentially improve their thermal conductivity and stability in demanding environments, leading to enhanced performance. However, there's a long road to see this become a reality.
3. The ability to exploit quantum entanglement in phase shifter control systems could enable parallel calculations at incredibly high speeds. This could create real-time responses to dynamic changes within supercritical phase-shifting environments, leading to potentially more efficient processes. But can it truly work as well as the theory suggests?
4. While it's an exciting idea, integrating quantum computing isn't necessarily a straightforward path. Certain types of quantum hardware, like superconducting qubits, face unique difficulties due to their need for extremely low temperatures. This could make integration into existing systems tricky.
5. Quantum computing's inherently probabilistic nature creates both opportunities and challenges. While it can explore a huge range of potential solutions for phase shifter optimization quickly, it also needs robust error correction mechanisms to ensure accurate results. This increases system complexity.
6. Emerging research suggests that unique quantum superposition states could be leveraged to create entirely new types of phase-shifting materials. This could lead to significant breakthroughs that might push antimatter production efficiency beyond what's currently achievable. It's a fascinating theoretical concept, but are there feasible paths to achieve it?
7. When you integrate quantum computing with phase shifter control systems, it highlights the critical role of error rates. Even small inaccuracies in measuring quantum states can cause considerable deviations in the expected behavior of phase shifters, potentially affecting system reliability. We'll need very careful attention to this.
8. Introducing quantum-enhanced sensors into phase shifter systems could potentially greatly improve the accuracy of temperature and pressure readings. These measurements are vital for maintaining stability and optimal conditions for antimatter production. It's an area ripe for innovation.
9. However, bringing quantum computing into existing setups isn't without its hurdles. The need to maintain a consistent, very low-temperature environment poses a substantial challenge. This requires carefully designed cooling strategies that work in concert with the control systems—a tough engineering problem.
10. Utilizing quantum simulations to model how antimatter behaves during phase shifting could reveal previously unseen phenomena. This might give us a better understanding of unusual states of matter crucial to antimatter production, opening up new avenues of research. This is a potentially very fruitful area.
Optimizing Antimatter Production A Technical Analysis of Supercritical Phase Shifter Power Requirements in AI-Driven Systems - Engineering Solutions for Dark Matter Containment Field Stability
Within the broader context of optimizing antimatter production, the challenge of maintaining stable dark matter containment fields is a crucial engineering problem. Current methods for containing antimatter primarily rely on magnetic and electrostatic fields to prevent its annihilation with normal matter. This approach, while conceptually sound, faces significant hurdles. Traditional methods have demonstrated limited efficiency, hindering their practicality for widespread use, especially in applications like space propulsion where scaling becomes critical. Moreover, the inherent risks associated with managing polonium gas, a key component in many antimatter production schemes, add another layer of complexity. Its high radioactivity necessitates robust containment and necessitates careful attention to material selection and radiological safety.
The path forward requires a multi-pronged approach that integrates the latest advancements in related fields. The potential for quantum computing to model complex interactions within the containment field, and its capacity to inform the development of novel materials, is a particularly exciting prospect. These combined efforts are crucial for ensuring that future dark matter containment systems are both stable and safe enough to facilitate exploration of antimatter’s potential across a variety of applications. Despite the challenges, this pursuit represents an area of crucial development for the continued progress of antimatter research.
Engineering solutions for stabilizing dark matter containment fields are built on the idea that dark matter primarily interacts with regular matter through gravity. This presents a unique engineering challenge because it requires finding ways to create electromagnetic barriers that can manipulate these elusive particles.
The search for stable dark matter containment fields has led researchers to explore metamaterials—engineered materials with properties not seen in nature. These materials could be designed to affect how electromagnetic waves travel, which could indirectly help stabilize areas where dark matter might be concentrated.
One fascinating aspect of working with dark matter is the potential to use quantum coherence to stabilize containment fields. By aligning the quantum states of particles, scientists hope to create stronger containment environments that can resist the effects of outside gravitational forces.
Recent studies suggest that changes in the amount of dark matter surrounding containment fields could affect their stability. Engineers are exploring adaptive systems that can automatically adjust themselves based on real-time data about dark matter fluctuations to ensure the best possible containment.
The connection between dark matter and how gravity bends light (gravitational lensing) could be useful for designing containment systems. Techniques from gravitational lensing research might offer insights into how to create containment fields that focus or spread out dark matter more effectively.
Current approaches to engineering dark matter containment frequently face limitations in what we know about physics. Ideas like negative mass and exotic matter are being considered, but these concepts are mostly theoretical and not yet practical in real-world engineering.
The idea of using a plasma state in containment fields has opened up new research avenues. Plasma can be manipulated using magnetic and electric fields, offering a potential way to make dynamic barriers that might better stabilize areas of dark matter.
Engineering solutions for dark matter containment often need to manage the issue of energy loss. Research suggests that building a system that is both energy efficient and maximizes containment effectiveness requires innovative cooling systems that can adapt to high-energy demands.
Research into how dark matter interacts with other things suggests that adding artificial intelligence to monitoring systems could lead to groundbreaking advances in detecting changes in containment field stability. This could provide engineers with real-time feedback to prevent potential problems.
The possibility of incorporating nanotechnology into dark matter containment systems shows promise. Materials at the nanoscale could enhance the precision and strength of barriers designed to interact with dark matter, potentially overcoming some of the obstacles faced with larger-scale systems.
Optimizing Antimatter Production A Technical Analysis of Supercritical Phase Shifter Power Requirements in AI-Driven Systems - Material Science Breakthroughs in High Temperature Superconducting Coils
Recent advancements in the field of material science, specifically concerning high-temperature superconducting (HTS) coils, offer exciting possibilities for improving our energy infrastructure. HTS materials, unlike their low-temperature counterparts, can function efficiently at the relatively warmer temperatures achievable with liquid nitrogen, typically between 65K and 80K. This temperature range makes HTS systems more practical for various applications, including the crucial field of power transmission.
The emergence of second-generation (2G) HTS materials, such as those based on rare earth barium copper oxide (REBCO) coated conductors, is particularly promising. These materials are manufactured with innovative techniques, leading to better performance and reliability in a range of energy-related applications. These improvements are leading to increased interest in applications such as nuclear fusion, which requires incredibly powerful magnets, and the developing field of wireless power transmission for electric vehicles.
While HTS technology shows immense potential, there are also challenges that need to be addressed for widespread adoption. One significant concern involves minimizing alternating current (AC) losses within HTS coils, which can impact their efficiency. Ongoing research aims to overcome these obstacles and continue to expand the range of applications where HTS can be effectively implemented. Ultimately, these advancements could play a pivotal role in the global transition toward a carbon-free energy future.
The progress in HTS technology is a strong example of how material science breakthroughs can address critical energy needs. This intersection of materials research and energy technology is likely to remain vital as we seek solutions for the increasingly complex energy challenges that face our world.
High-temperature superconducting (HTS) coils offer a compelling pathway to improving the efficiency of antimatter production systems, especially when compared to traditional magnetic systems. These coils can carry electricity without resistance even at temperatures well above the boiling point of nitrogen (77K), potentially leading to more efficient electromagnets. Recent innovations in HTS materials, particularly those incorporating rare-earth elements, have led to substantial gains in the amount of current they can handle, allowing for the creation of smaller and more powerful coils. This is especially attractive for applications like spacecraft propulsion, where minimizing the weight of equipment is crucial.
The discovery of superconducting materials that function at temperatures closer to -135 degrees Celsius (138K) is a major step towards potentially realizing HTS technologies in wider applications, including power grids. It's a notable achievement, though the transition from lab to practical use remains a challenge. Intriguingly, researchers are finding that certain defects in the structure of HTS materials can actually improve their superconducting behavior, contradicting prior assumptions. This discovery suggests a new approach to designing these materials.
These coils are capable of sustaining very strong magnetic fields, exceeding 10 Tesla, which is critical for achieving the necessary conditions for antimatter production and containment. Advances in manufacturing, specifically the development of coated conductor technology, have led to high-quality HTS wires that can be used to build even better coils. When combined with efficient cooling techniques, HTS coils show great potential for reducing the energy needs of maintaining superconductivity within antimatter production setups.
Computer simulations suggest we can control the strength of the magnetic field produced by HTS materials by carefully adjusting their internal structure. This has provided valuable insights for designing HTS coils that are ideal for specific tasks in antimatter systems. The financial implications of utilizing HTS technologies are substantial. While the upfront investment in HTS systems is typically high, the potential reduction in operational costs and the increase in efficiency of antimatter production processes creates a strong case for continued exploration and development of these promising materials. There are still hurdles to overcome, but the potential benefits appear to outweigh the challenges in the longer term.
More Posts from aistructuralreview.com: