Analyzing Supersonic Flow Characteristics A Deep Dive into Modern Prandtl-Meyer Calculator Applications in Aircraft Design
Analyzing Supersonic Flow Characteristics A Deep Dive into Modern Prandtl-Meyer Calculator Applications in Aircraft Design - Prandtl-Meyer Wave Functions Mathematical Framework Behind Modern Aircraft Wing Design
The foundation of modern supersonic aircraft wing design relies heavily on the Prandtl-Meyer wave functions. These functions are essential for understanding how supersonic airflow behaves when it turns around a corner, a crucial aspect for wing design. The core concept revolves around the change in Mach number as the flow expands, a process that is isentropic, meaning there's no increase in entropy. This is a key distinction from shock waves, which are not isentropic. The Prandtl-Meyer function allows engineers to calculate how much the Mach number will change for a given angle of the turn. Using this, they can refine wing shapes to achieve the desired aerodynamic performance.
Furthermore, these functions are utilized in complex numerical analyses of supersonic airfoils. This helps in accurately understanding the forces and moments exerted on the aircraft, offering a pathway for improved performance under supersonic conditions. Essentially, the Prandtl-Meyer function serves as a mathematical lens into supersonic aerodynamics, helping design supersonic aircraft components that are both efficient and effective. It’s a crucial tool in the ongoing development and evolution of aerospace technology.
When supersonic airflow encounters a curved surface, it doesn't simply compress or deflect; it expands in a characteristic fan-like pattern, known as the Prandtl-Meyer expansion fan. This expansion, unlike shock waves, happens without an increase in entropy, making it an isentropic process. The core idea is captured by the Prandtl-Meyer function, a tool allowing us to connect the angle of the turn with the resulting changes in the Mach number. The Prandtl-Meyer angle, in essence, represents the extent to which a supersonic flow expands to reach a particular Mach number.
The fundamental connection between the flow deflection and the related changes in Mach number stems from the basic principles of mass and momentum conservation across the expansion fan. The Prandtl-Meyer function itself embodies this relationship, linking initial and final Mach numbers while factoring in specific heat capacities.
Understanding these aerodynamic characteristics, especially the pressure distribution on the wing surface, becomes crucial for designing supersonic airfoils. The Prandtl-Meyer function provides that understanding. Modern computational tools can handle the often complex calculations associated with the function, significantly easing the design and analysis of supersonic aircraft components.
In essence, the Prandtl-Meyer function acts as a foundational tool within modern aircraft wing design, particularly when focusing on optimizing performance at supersonic speeds. It's worth noting that the method of characteristics, another important analytical tool, is often used alongside the Prandtl-Meyer function to provide deeper insight into the nuances of supersonic flows, which involve these expansion fans.
While this framework provides valuable insights, it's crucial to recognize that its application can be challenging for complex wing geometries. Real-world flows can be much more complex than simplified models used in calculations, leading to a potential gap between theory and practice. The development of refined computational methods is therefore crucial to enhance the accuracy of Prandtl-Meyer function applications for intricate geometries.
Analyzing Supersonic Flow Characteristics A Deep Dive into Modern Prandtl-Meyer Calculator Applications in Aircraft Design - Flight Testing Data Analysis From X-59 Quiet Supersonic Transport Integration With Flow Models
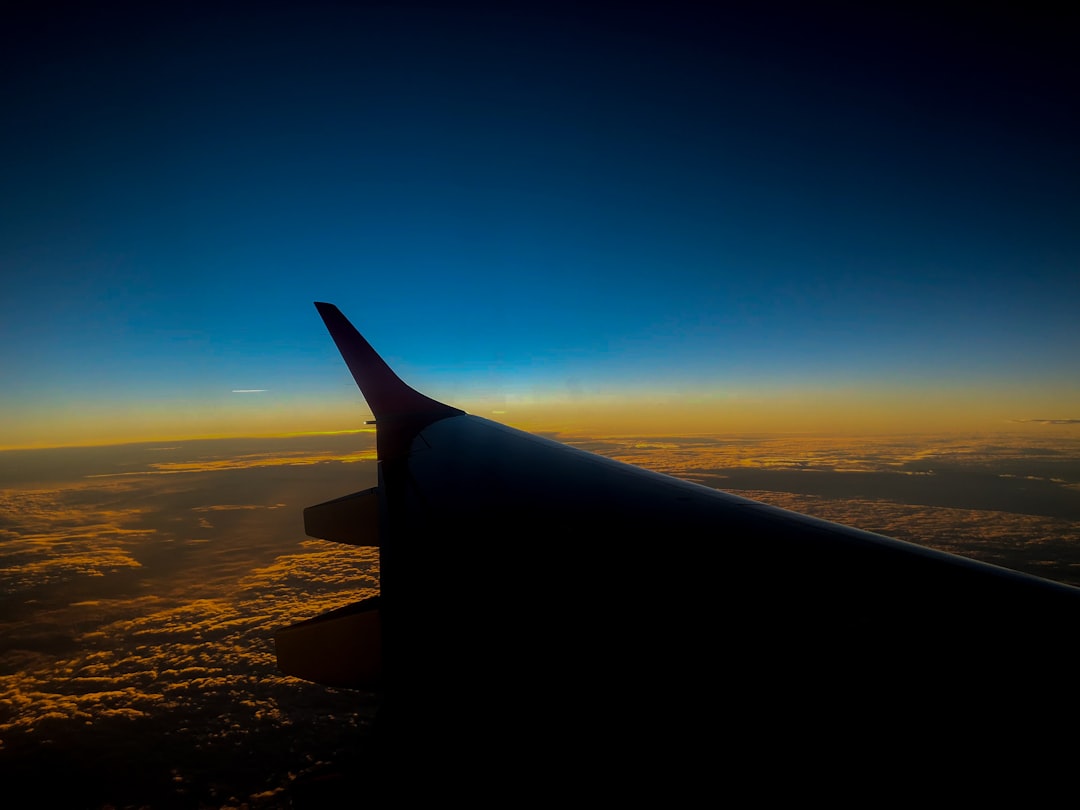
The X-59 Quiet Supersonic Transport program is pushing the boundaries of supersonic flight by focusing on minimizing the disruptive sonic boom. Through flight testing at approximately Mach 1.4, the X-59 is gathering valuable data on community response to the aircraft's noise profile. This data is critical for influencing future regulations and guiding the design of quieter supersonic aircraft. It's a balancing act between technological achievement and public acceptance. In parallel, NASA's supercomputing resources are being used to create precise models of the sonic boom generated by the X-59. These models will be essential for ensuring the aircraft can operate safely and quietly over populated regions. The ultimate goal is to change how we view supersonic flight, potentially paving the way for commercially viable supersonic travel that doesn't disrupt communities on the ground. While there are challenges in accurately simulating complex airflow, the X-59 project represents a significant leap forward in exploring the possibilities of quieter supersonic transport. The success of the X-59's flight testing and data analysis efforts could have a profound impact on the future of aviation.
NASA's X-59 Quiet Supersonic Transport (QueSST) program is a fascinating endeavor aimed at understanding and ultimately mitigating the loud sonic booms associated with supersonic flight. The aircraft is being meticulously tested, not just for its structural integrity, but also to collect valuable data on how airflow behaves at these high speeds. It's particularly interesting how the integration of various flow models into the flight test program is helping us to better understand the complexities of supersonic flow, especially how the transition from subsonic to supersonic speeds impacts the entire system.
We're learning a lot about the intricate interplay between the X-59's innovative engine placement and the resulting airflow characteristics. The data collected is, in a sense, a practical validation of established theories like Prandtl-Meyer expansion waves, which we've previously discussed. This data shows us how these expansion fans influence shock wave patterns in a real-world context.
The ability to collect data across a broad spectrum of Mach numbers is incredibly valuable. This gives us a much deeper insight into the transitional regions of supersonic flight, areas that are often less understood. What's especially useful is the real-time visualization of airflow during flight. This provides engineers with instantaneous feedback on the effectiveness of the various design choices.
However, it's important to highlight some of the unforeseen challenges the researchers encountered. Flight tests have revealed that flow separation phenomena become more prominent at higher angles of attack, indicating potential complications in specific flight conditions.
Further analysis of this unexpected behavior will likely be needed for refining the design and optimizing safety. The combination of computational fluid dynamics (CFD) simulations and real flight data is helping to refine the aerodynamic models and validate the predictions against actual aircraft performance. The development of cutting-edge sensors on the X-59 is allowing for a much finer level of data capture, including pressure, temperature, and turbulence intensity, giving a level of detail that was previously unavailable.
In essence, the X-59 is a remarkable testbed for validating the design concepts that are being developed for future generations of supersonic aircraft. It's showing us that with thoughtful design and careful consideration of the related physics, it is possible to achieve significant performance improvements in supersonic flight. However, it’s equally apparent that the path towards quieter and more efficient supersonic travel is still fraught with challenges that require persistent investigation and innovation. The X-59’s role is not just to prove concepts, but also to refine design in a way that paves the path for a safer and more environmentally conscious approach to supersonic flight.
Analyzing Supersonic Flow Characteristics A Deep Dive into Modern Prandtl-Meyer Calculator Applications in Aircraft Design - Temperature Distribution Patterns During Supersonic Flow Across Variable Cross Sections
Supersonic flow traversing variable cross-sections, such as within nozzles, experiences temperature distributions that are heavily influenced by the nozzle's shape, especially in the region after the throat where the flow speeds up. The way the nozzle is shaped can have a big impact on the flow, with factors like the Prandtl-Meyer angle being particularly important. If the nozzle isn't designed correctly, it can create unwanted shock waves and expansion waves, which can negatively affect performance.
Research has shown that in supersonic flows with turbulence, the temperature recovery factor generally falls between 0.875 and 0.895. This highlights the need for careful thermal management in the design of aerodynamic components. We also need a deeper understanding of how compressibility influences turbulence, particularly its effect on average temperature and density. Improving the accuracy of models in this area will lead to better predictions of thermal performance and efficiency in supersonic flows. Advanced computational methods become crucial in capturing these complex flow behaviors, particularly in the context of supersonic aircraft design. Without them, it's difficult to accurately predict these intricate interactions.
Supersonic flow through varying nozzle shapes leads to complex temperature patterns. The way the nozzle is designed, especially after the narrowest part (the throat) where the flow speeds up to supersonic speeds, significantly affects how the temperature and Mach number change.
It's noteworthy that abrupt changes in the Prandtl-Meyer angle, which describes how much the flow expands around a corner, can result in unwanted shock waves and expansion waves. This highlights the crucial role of precise nozzle design in supersonic applications.
Researchers have conducted experiments involving turbulent heat fluxes to gather reliable data about the speed, temperature, and density of air inside round nozzles that widen and then narrow (convergent-divergent nozzles). Some of this foundational work dates back nearly 30 years, showcasing the longevity of these challenges.
The Prandtl-Meyer function is vital in understanding how supersonic airflow changes in a predictable way (isentropically). It helps us see how things like lift and drag change on supersonic airfoils, important for aircraft design.
When we examine supersonic flows with turbulence, the changes in density and temperature, along with thermodynamic fluctuations, affect the flow near the walls. This is another layer of complexity added to already complex conditions.
Visual studies of supersonic airflow reveal a phenomenon where the leading edge of an airfoil can separate from the flow at higher angles of attack, potentially creating vortex-like structures that negatively impact performance.
Interestingly, measured temperature recovery factors (a measure of how much heat energy is transferred into the surface) in supersonic turbulent flows fall within a specific range (0.875 to 0.895). This information is a key part of managing heat loads on aerodynamic parts.
There are some fascinating things to observe in the heat transfer during supersonic flows. For instance, the stagnation temperature (the temperature of the air at a point where the flow is brought to rest) and the initial Mach number of the flow have direct consequences for overall thermal performance.
Modeling temperature distribution accurately within supersonic turbulent boundary layers is a significant challenge. This aspect is critical to optimize both thermal management and aerodynamic performance in aircraft design.
Integrating modern Prandtl-Meyer calculators into the aircraft design process is incredibly beneficial for engineers. These tools provide sophisticated means for examining complicated airflow patterns and consequently improve the aerodynamic performance of aircraft. It's a clear indication of how the field has advanced.
Analyzing Supersonic Flow Characteristics A Deep Dive into Modern Prandtl-Meyer Calculator Applications in Aircraft Design - Real Time Flow Monitoring Systems Applications In Wind Tunnel Testing 2024
Real-time flow monitoring systems are becoming increasingly vital in wind tunnel testing, acting as a bridge between theoretical models and practical observations. They allow engineers to validate computational fluid dynamics (CFD) simulations in real-world settings, creating a richer understanding of how air flows around objects. Methods like flow visualization, for instance, provide a detailed look at complex aerodynamic interactions, leading to improved understanding of aerodynamic performance, especially crucial when dealing with supersonic aircraft.
The field is also witnessing the adoption of modern techniques such as machine learning and physics-informed reconstructions within these real-time monitoring systems. These new tools offer greater potential for manipulating and controlling airflow under turbulent conditions, a significant challenge in aviation. These changes in how flow is monitored are not just improving our knowledge of the fundamentals, but they're also changing how we design aircraft. The ability to see and adjust the airflow in real time is allowing for more agile optimization of aircraft performance across various flight regimes. As we move forward, it's likely that real-time flow monitoring systems will be fundamental to the design of even more efficient and effective aircraft.
Real-time flow monitoring systems are becoming more common in wind tunnel tests, primarily to assess the accuracy of computational fluid dynamics (CFD) tools and to provide a better understanding of experimental results. These systems allow for a continuous stream of data that can reveal details about the flow not captured by older methods. For example, the NASA Ames Unitary Plan Wind Tunnel (UPWT) uses flow visualization techniques, like schlieren photography, to study external flows, allowing researchers to better grasp aerodynamic behavior around test models.
Historically, wind tunnel testing relied heavily on techniques like measuring force and momentum changes to estimate an aircraft's aerodynamic performance, producing data represented as polar plots for airfoils. Now, there's a shift towards more advanced methods. These newer techniques, including methods based on physics and machine learning, are being integrated into real-time flow monitoring, enhancing the possibility of dynamically controlling airflow under a variety of atmospheric conditions.
The 8x6 Supersonic Wind Tunnel at NASA Glenn Research Center, notable as the only transonic propulsion wind tunnel spanning Mach 0.26 to 2.0, highlights the need for this type of flow monitoring. For instance, a recent study by the Dufour Aerospace Flight Physics team used this facility to investigate tilt-wing aircraft aerodynamics. This demonstrates the importance of wind tunnels in aircraft development, and, arguably, the potential need to upgrade these facilities with real-time flow monitoring capabilities for a wider variety of testing conditions.
On the other hand, a large low-speed closed-circuit wind tunnel at the Norwegian University of Science and Technology (NTNU) shows how versatile wind tunnel testing can be, even without supersonic capabilities. Equipped with a 220 kW fan, it's capable of producing winds up to 30 m/s, making it a good resource for testing wind turbine performance, among other low-speed applications. This showcases how wind tunnel technology is adaptable across a broad spectrum of research.
While supersonic wind tunnel tests remain crucial for analyzing supersonic airflow behavior, it's worth noting that challenges remain. In the realm of high-speed flight, accurately predicting the interaction of supersonic flows with aircraft structures is essential for optimizing performance, and, in this respect, the Prandtl-Meyer calculator has become a valuable tool. The ability to perform more precise calculations on supersonic flow transitions and shock wave behaviors has further improved the design capabilities for engineers. This is helpful when considering the challenges related to high-speed flows, such as those associated with shock wave behavior. The further development of such computational tools is a vital element of modern aircraft design.
However, challenges remain. Real-world aerodynamic problems are often incredibly complex, far exceeding simplified models that can be analyzed computationally. This creates some disconnect between what can be theoretically calculated and what actually occurs in reality. Ultimately, the accuracy of these computational tools is reliant on being able to improve experimental methods that capture the flow behavior, and it's in this area where the real-time flow monitoring systems might play an increasing role.
Analyzing Supersonic Flow Characteristics A Deep Dive into Modern Prandtl-Meyer Calculator Applications in Aircraft Design - Shock Wave Boundary Layer Interactions Impact On Modern Supersonic Aircraft Design
In the realm of supersonic aircraft design, understanding the impact of shock wave boundary layer interactions (SWBLI) is paramount for achieving optimal performance and safety. These interactions, which occur when shock waves encounter the boundary layer of air along the aircraft's surface, can lead to a variety of undesirable consequences. One major issue is the thickening of the boundary layer itself, which can interfere with the smooth flow of air over the aircraft's surfaces. This can also lead to flow separation, where the airflow detaches from the surface, potentially causing instability and reducing aerodynamic efficiency.
Furthermore, SWBLI can contribute to phenomena like inlet buzz, where pressure oscillations within the aircraft's engine inlet cause unsteady and potentially damaging conditions. In severe cases, this can lead to inlet unstart, a complete disruption of airflow into the engine that can cause a loss of thrust. These effects are especially pronounced in the three-dimensional flow fields found around many supersonic aircraft components, such as inlets, nozzles, and control surfaces.
Given the complexity of these interactions, engineers rely on both passive and active control techniques to mitigate their negative impact. These methods seek to manage the development of shock waves and boundary layers in a way that enhances aerodynamic performance and aircraft reliability. As supersonic aircraft designs become increasingly sophisticated, a deep understanding of SWBLI is needed to ensure that these interactions are accounted for throughout the design process. Failing to address these challenges could compromise performance, safety, and overall efficiency.
Ultimately, the successful development of next-generation supersonic aircraft hinges on the ability to effectively model and manage SWBLI. This requires incorporating advanced analyses that consider the complex geometries and shock wave configurations encountered in practical applications. Only through a deeper understanding of these phenomena can engineers effectively optimize aircraft performance and ensure the safe and efficient operation of these high-speed machines.
Shock wave boundary layer interactions (SWBLI) are a major factor in the design of supersonic aircraft. The location and strength of shock waves have a direct impact on the drag and stability of an aircraft, and misjudging these interactions can cause performance problems that could compromise safety and efficiency.
A particularly important aspect is shock-induced boundary layer separation. This occurs when the boundary layer separates from the aircraft's surface, which leads to increased drag and potential control issues. Engineers must carefully design surfaces to minimize this effect during high-angle-of-attack maneuvers.
SWBLI can create complex pressure fields that result in unexpected changes in the aerodynamic forces, like lift and drag. This underscores the importance of accurate prediction and modeling of these interactions during the design process.
The transition from laminar to turbulent flow (boundary layer transition) in the boundary layer also plays a key role in the interactions with shock waves. This transition can alter the characteristics of shock waves, affecting both the aerodynamic performance and heat transfer into the aircraft's structure.
Computational fluid dynamics (CFD) has become crucial for understanding these intricate interactions. While CFD simulations are able to capture fine flow features that traditional methods miss, they are demanding in terms of computational resources and rely on rigorous validation using real-world data.
Intriguingly, SWBLI can also increase noise generation in supersonic aircraft, which needs to be carefully considered due to community noise regulations and acceptance. Understanding the physics of these interactions is key to reducing the associated noise.
The design of supersonic aircraft frequently requires compromises. While minimizing drag is a primary goal, some design choices that lead to strong shock waves can also improve stability and control during certain flight regimes. This highlights the intricate balancing act that engineers need to perform during the design process.
Researchers are exploring innovative materials and coatings to better manage the significant heat loads produced by SWBLI. This thermal management is critical given the extreme temperatures encountered during supersonic flight.
The optimization of wing shapes using tools like the Prandtl-Meyer function becomes especially important for managing SWBLI because small changes in wing shape can have a big impact on the expansion or compression of air around crucial surfaces.
Finally, studies of SWBLI increasingly involve experiments like wind tunnel testing, where real-time flow monitoring systems are used to capture rapidly changing flow behavior. The information gleaned from these systems then contributes directly to the iterative design process for supersonic aircraft development.
Analyzing Supersonic Flow Characteristics A Deep Dive into Modern Prandtl-Meyer Calculator Applications in Aircraft Design - Machine Learning Algorithms In Supersonic Flow Pattern Recognition And Analysis
Machine learning is becoming increasingly important in understanding supersonic flow patterns. It offers new ways to analyze complex flow behaviors at high speeds. Algorithms like support vector machines help predict how two-phase flows behave, which is vital for managing heat and designing efficient propulsion systems. Deep learning approaches are also being explored to reconstruct flow fields quickly and accurately. Interestingly, there's a developing trend to integrate physics-based ideas with deep learning, creating what are called "physics-informed neural networks." These hybrid methods could provide more accurate real-time monitoring and control of supersonic airflow. As we continue to develop supersonic aircraft, these machine learning methods could be valuable tools for tackling tough challenges such as shock waves that interact with the boundary layer of air around the aircraft. These interactions can affect things like stability, efficiency, and safety. The potential for machine learning to optimize aircraft design and advance supersonic aviation is quite promising. However, it's important to remember that many of these techniques are still relatively new and are constantly evolving. The challenges presented by supersonic flows are also extremely difficult. Despite these challenges, machine learning has the potential to significantly impact this field.
Machine learning (ML) offers some intriguing approaches to analyzing supersonic flow data, potentially enhancing our comprehension of the underlying mechanisms. For instance, support vector machines (SVMs) have been explored for predicting two-phase flow patterns, a crucial aspect of reactor safety in some novel nuclear power designs. The use of ML in this field suggests the ability to predict behaviors where otherwise only highly detailed simulations were previously practical, which is quite promising.
In practical supersonic applications, like designing supersonic separators, the placement of vortex generators significantly influences flow characteristics, as shown by studies of the 3S and BV twister models. This is an interesting observation because it suggests that machine learning could help with the optimization of features, such as vortex generators, which is an area that previously was often approached more through experiment and testing, especially with the unique constraints that come with supersonic flows.
The emergence of frameworks like the supersonic physics-constrained network (SPC) hints at a growing trend of merging deep learning with physics-based approaches. One can only wonder if, using the power of computation, engineers could use SPC in conjunction with existing theories like Prandtl-Meyer to resolve some of the complexity related to non-ideal flows that exist in the real world. This certainly appears to be a very promising area of research, and potentially a very useful area for future aircraft design where accuracy in modeling has a crucial impact on safety.
Supersonic engine inlets are a particularly critical area where flow pattern recognition can improve protection and control. Hybrid algorithms combining fast K-nearest neighbors (FKNN) and improved directed acyclic graph support vector machine (IDAGSVM) have been proposed to enhance this capability. This makes sense, as this type of problem is probably ideal for an application of machine learning techniques, as it's one where the number of potential variables can easily lead to a situation where traditional techniques struggle.
Flow pattern predictions in two-phase systems are influenced by a range of factors, including liquid and gas velocities, viscosities, densities, and surface tension. The complexity of these variables highlights the need for sophisticated tools, including machine learning, to provide comprehensive solutions in these types of challenging scenarios.
Deep learning models have also been suggested for reconstructing flow fields in supersonic cascades, enabling swift and accurate flow state monitoring. Again, this makes intuitive sense, as these types of complex flows are often characterized by a large number of intertwined variables that are difficult to isolate when applying older techniques of analysis. It does suggest that we will continue to see progress in these areas.
Extracting patterns from massive datasets associated with supersonic flow characteristics is paramount for active flow control applications. This is where the power of ML can be particularly helpful. The design of supersonic flow separators illustrates this quite well, where the positioning of rotating vanes significantly impacts the resulting patterns. It would be interesting to see this type of model deployed in the context of aircraft design as this has the potential to change how components are designed and potentially improve the robustness of certain designs.
The development of ML techniques in recent years has emphasized their applicability in real-time monitoring and control of supersonic flow environments. This ability to create more accurate flow model predictions suggests that we will continue to see increasingly sophisticated applications of ML techniques and that we will also see an increasing integration of these tools into wind tunnel testing.
Despite their considerable potential, there are also challenges and limitations associated with the application of ML in the area of supersonic flows. ML algorithms are often developed for specific flow regimes and can sometimes struggle to generalize these results to different or more complex scenarios, which means that extensive verification will likely remain a requirement when applying these tools. The complexity of some supersonic flow regimes is inherently nonlinear, which is known to challenge some machine learning applications. Thus, the need for continual development and refinement of these algorithms is unavoidable.
More Posts from aistructuralreview.com: