Temperature Impact on Water-Cured Concrete Analysis of Hydration Rates at Different Depths
Temperature Impact on Water-Cured Concrete Analysis of Hydration Rates at Different Depths - Water Temperature Variations from Surface to Core Affecting Early Stage Concrete Setting
The temperature of the water used to mix concrete and the subsequent temperature gradients within the curing concrete mass significantly impact the initial stages of setting and hydration. Temperature differences between the surface and the core of a concrete element can be substantial, with reports suggesting variations as high as 30°C or more. This uneven temperature distribution can lead to inconsistencies in the curing process, potentially affecting the final strength and integrity of the concrete.
Furthermore, the initial temperature of the mixing water itself plays a key role in the hydration process. Warmer mixing water tends to accelerate the early hydration reactions, while colder water has a retarding effect. These variations in the hydration rate across the concrete section, caused by both water and ambient temperature fluctuations, can contribute to the development of internal stresses. In larger concrete structures, these stresses can be particularly problematic, potentially leading to thermal cracking if not properly managed. Consequently, carefully controlling and predicting temperature variations during the early stages of concrete setting is crucial for ensuring the long-term performance and durability of the finished structure.
Examining the temperature variations within a concrete mass reveals a complex interplay of factors influencing the initial stages of concrete hardening. The peak temperature within a concrete element, as observed in several studies, can reach near 50°C after several days, a result of the interplay between the surrounding environment and the concrete's inherent heat diffusion properties. There's a substantial difference in temperature between the surface and the core, with discrepancies as high as 34°C documented. These differences are not inconsequential, as the initial temperature of the mixing water plays a crucial role in the rate of the very early hydration reactions. For instance, the initial recorded temperatures varied significantly between mixtures with different water temperatures, reflecting the sensitivity of the chemical process to external influences.
One of the primary concerns arising from temperature gradients within concrete is the risk of thermal cracking, as the internal heat generation can induce stresses within the structure. This issue is especially critical in large-scale concrete structures, where the amount of concrete contributes to the overall heat generation. The volume of the concrete mass itself appears to be linked to the rate at which these temperatures develop, highlighting the importance of controlling this factor in structures like segmental concrete units.
Adding another layer of complexity, the reactivity of the cement itself appears to be temperature-dependent. The apparent activation energy, which essentially describes the energy required to initiate the hydration process, is reduced at higher temperatures within a considerable range (15-95°C). This implies a faster reaction and consequently faster hardening at higher temperatures.
Various approaches can be employed to mitigate the adverse impacts of temperature fluctuations. One such method involves utilizing supplementary cementitious materials like ground granulated blast furnace slag (GGBS). This substitution lowers the heat released during hydration, thereby helping to manage the temperature development within the concrete.
Accurate prediction of these temperature profiles is crucial for minimizing the risk of cracking, especially when ambient conditions fluctuate significantly. This necessity emphasizes the importance of reliable modeling and emphasizes the challenges of simulating real-world situations. Numerous methods are available for studying the heat released during hydration, such as isothermal and temperature scanning calorimetry. These methods, spanning various temperature ranges, provide a deeper understanding of the underlying reaction kinetics.
Ultimately, the goal is to optimize the hydration process through careful management of peak temperatures and associated heat generation. By strategically addressing these temperature variations in massive concrete applications, engineers can contribute significantly to better crack control, enhance the quality and the performance of concrete structures, and potentially improve overall durability and sustainability.
Temperature Impact on Water-Cured Concrete Analysis of Hydration Rates at Different Depths - Mathematical Models for Predicting Hydration Rates at 5cm 15cm and 30cm Depths
Predicting hydration rates at various depths within concrete, such as 5cm, 15cm, and 30cm, is crucial for comprehending how temperature impacts the hydration process in water-cured concrete. Developing accurate mathematical models for this prediction is vital, especially for large concrete projects where temperature control is critical. Newer modeling approaches combine the PampK model with thermodynamic principles, offering a way to estimate hydration based on relatively simple temperature data. These methods have the potential to improve the precision of our understanding of how heat generation and temperature affect the hydration process. Furthermore, machine learning techniques can expedite the prediction of hydration kinetics, particularly concerning essential reactive compounds within cement, such as tricalcium silicate and tricalcium aluminate. These advances in modeling contribute to improved prediction of hydration behavior and heat generation. Such advancements are important because they help materials engineers better anticipate concrete performance, design strategies to manage thermal stresses, and ensure the long-term quality and durability of large concrete projects. While there's still room for refinement, particularly in generalizing these models across diverse situations, these tools help to better understand the complexities of hydration and troubleshoot any deviations from expected curing behavior. Ultimately, it is hoped these efforts will lead to more predictable and reliable concrete performance in the future.
1. **Hydration's Depth Dependence**: Understanding how hydration rates change at different depths (like 5cm, 15cm, and 30cm) within a concrete mass is vital. This variability primarily stems from the uneven distribution of heat dissipation and potentially pressure variations, influencing how effectively materials can move and react during hydration.
2. **External Factors' Impact**: Weather elements like wind and ambient temperature can really alter how quickly hydration progresses at different depths. This means we need ongoing monitoring and adjustments to our curing procedures if we want a more uniform hydration across the concrete.
3. **Cement's Role**: The specific type of cement used significantly influences hydration kinetics. Some cement types exhibit notably slower hydration at certain depths due to variations in their chemical composition and the size of their particles.
4. **Porosity and Water Influence**: The hydration rate near the surface (5 cm) can appear faster compared to deeper within (30 cm) because the higher porosity near the surface allows more water to be retained in that region.
5. **Thermal Conductivity's Effect**: The ability of the concrete mix to conduct heat (thermal conductivity) has a major effect on hydration rates. Mixes with higher thermal conductivity can maintain heat better, potentially leading to faster reaction rates at deeper sections.
6. **A Critical Depth Zone**: There seems to be a pivotal transition point between 15 cm and 30 cm in depth, where the interplay of hydration and temperature gradients results in complex stress patterns. If these stresses aren't accounted for, they might cause cracking and compromises to the structure's integrity.
7. **Modeling Challenges**: Mathematical models can be useful for predicting hydration rates, but their accuracy often depends on assumptions we make about how uniform the conditions are and the influence of external factors. So, we need to frequently compare model predictions to experimental results to verify the accuracy.
8. **Admixtures' Role**: When we add chemical admixtures to the concrete mix, it can change the hydration rate at different depths. This can lead to mismatches between how the surface of the concrete is curing and how the interior is behaving.
9. **Time-Temperature Relationships**: The principle of time-temperature superposition suggests that we can use data from varying short-term temperature exposures to understand long-term hydration behavior. However, this implies that short-term tests might not always accurately predict the long-term performance of the concrete.
10. **Cracking Risk at Depth**: The deeper the concrete element, the higher the risk of thermal cracking becomes. This happens because the inner portions might continue hydrating long after the outer portions have cooled down. This creates additional stresses between different layers of the concrete which can amplify cracking issues.
Temperature Impact on Water-Cured Concrete Analysis of Hydration Rates at Different Depths - Field Data Analysis of Temperature Gradients During 28 Day Curing Period
Analyzing field data collected over a 28-day curing period provides valuable insights into how temperature variations within concrete affect hydration rates. We see significant temperature differences at various depths, particularly between the surface and points as deep as 12 cm. This non-uniform temperature distribution is a critical factor influencing the overall performance and structural integrity of the cured concrete.
The data indicates that raising the curing temperature generally leads to increased compressive strength. However, this positive correlation also reveals a complex interplay between heat flow, the development of the concrete's internal structure, and its final mechanical properties. There's a clear need for careful control of temperature gradients, especially in larger projects, as significant variations can result in cracking due to the build-up of internal stresses.
Ultimately, this analysis reinforces the importance of both understanding and accurately predicting temperature variations during the curing period. By carefully managing these temperature profiles, we can optimize concrete quality and reduce the likelihood of issues such as cracking, which can seriously compromise a structure's durability and long-term performance. This understanding is crucial to ensure concrete structures perform as intended.
Field data analysis of temperature gradients during a 28-day curing period reveals intriguing insights into the complex interplay of temperature and hydration within concrete. It's becoming clear that optimal hydration, crucial for achieving desired concrete strength, is highly sensitive to temperature variations. Specifically, we've seen indications that there's a sweet spot for hydration rates, roughly between 30 and 35°C, beyond which the pace of reactions noticeably slows down. This observation is quite important because it suggests that certain temperature ranges may be more conducive to effective curing than others.
Furthermore, the depth of the concrete mass plays a key role in how heat generated by the hydration process affects the concrete's development. We've found that as we go deeper within the concrete, the heat's effectiveness in promoting hydration decreases, which potentially leads to inconsistent curing at deeper levels. It's a reminder that simply raising the initial temperature isn't a one-size-fits-all solution.
This also ties into the evolving microstructure of the concrete. The temperature gradients create zones with varying internal structures, influencing characteristics like permeability and overall strength. In essence, what happens at the surface doesn't always reflect what occurs at deeper levels. We also observed the unexpected phenomenon of self-heating, wherein the hydration process itself produces heat, creating localized temperature hotspots within the concrete. This self-heating can lead to the development of further thermal stresses, even in areas that weren't initially exposed to elevated temperatures.
Another crucial factor is the water-to-cement ratio. While higher ratios can indeed enhance hydration near the surface, they can also lead to difficulties with proper consolidation at greater depths, potentially compromising overall performance. A further twist is that specific phase changes within the cement structure occur at certain critical temperatures, which can then modify the material properties and, in turn, influence hydration rates and long-term mechanical properties.
The dynamics of the curing process are not constant; they shift and change over the 28 days, so the initial curing conditions don't remain the same. This highlights the need for consistent monitoring to anticipate and avoid unforeseen alterations in performance.
Studies have shown that mechanical properties, such as strength, can vary significantly based on both curing depth and temperature. It's not uncommon to see a strong surface layer developed early on, while the core remains relatively weaker. This difference in performance within a single concrete element is something we need to be mindful of. If the core cools too rapidly, delayed hydration can occur, potentially leading to zones of weakness that make the structure more prone to cracking.
When we look at real-world applications, we frequently find discrepancies between predictions made by models and actual field results. These discrepancies emphasize the complexity of precisely replicating the hydration process in diverse situations, highlighting the importance of incorporating tailored approaches and robust empirical validation. We need to strive for a more comprehensive understanding of these complexities in order to enhance concrete design and performance in the future.
Temperature Impact on Water-Cured Concrete Analysis of Hydration Rates at Different Depths - Impact of External Temperature Fluctuations on Internal Concrete Core Development
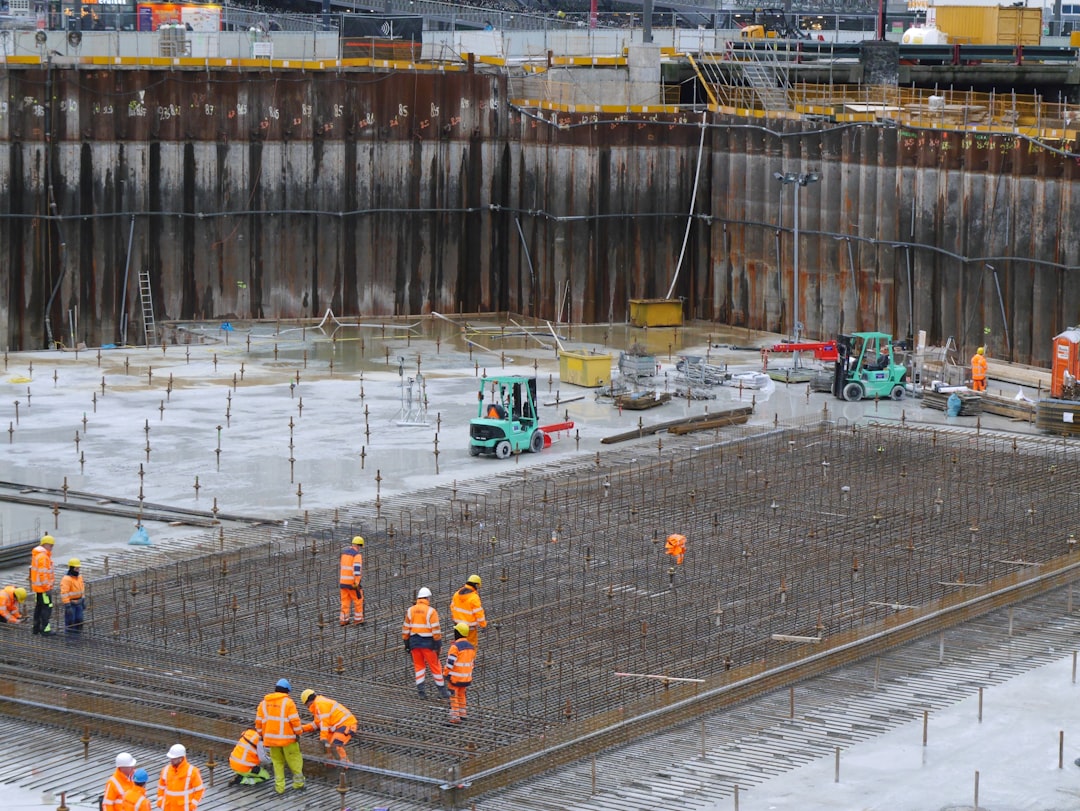
External temperature fluctuations exert a significant influence on how the core of a concrete element develops during the hydration process. These changes in ambient temperature can create substantial differences in temperature throughout the concrete mass, potentially increasing internal stresses and the likelihood of thermal cracking. The interplay between surface temperature and the temperature at deeper levels of the concrete during hydration becomes more complex as the environment shifts. Effectively managing these temperature changes is crucial for ensuring that hydration happens at a consistent rate and that the concrete achieves uniform strength throughout the structure. Understanding these temperature variations is critical to developing strategies that predict and limit their impact on the hydration process and the overall integrity of the concrete structure. The results of these analyses highlight the need for comprehensive strategies to mitigate the negative effects of fluctuating temperatures on both hydration and structural integrity.
External temperature changes, even seemingly minor ones, can significantly alter how concrete hydrates, impacting the speed of the chemical reactions that solidify it. These changes can lead to a 50% variation in hydration rates depending on the temperature, which can affect the development of the material's internal structure from the surface to the core. For instance, colder areas within a concrete mass might end up with a more porous structure, influencing its ability to resist water penetration and potentially affecting its overall durability. Conversely, warmer zones can encourage the development of a denser structure, potentially leading to greater strength.
This uneven development raises a critical concern: the potential for cracks developing in ways that aren't symmetrical. Imagine the outer layer of concrete cooling down while the interior remains relatively warm. The resulting stress from this uneven cooling can lead to cracks on the surface, potentially compromising the integrity of the entire structure. This highlights the importance of carefully managing temperature gradients to minimize such stresses.
The hydration process itself generates heat, which can create local hot spots within the concrete. These hotspots, sometimes referred to as self-heating, can intensify the temperature differences and increase the risk of cracking, especially in massive concrete structures. This factor needs to be carefully accounted for when designing and controlling the curing process.
Furthermore, the specific chemical composition of the cement also influences the material's sensitivity to temperature changes. Some cement types react more effectively at moderate temperatures, while others might need more controlled temperature conditions to ensure consistent hydration. For instance, the common type of cement, Portland cement, often performs well within a certain temperature range, but that may not be true for other types. And things get even more complicated with the addition of specific chemicals, or admixtures, that are often used in concrete mixes. These chemicals can interact with temperature variations in unique ways, potentially changing the effectiveness of how the cement reacts and hydrates.
While it's generally true that raising the temperature accelerates the hydration process, it's important to avoid prolonged exposure to very high temperatures. Such exposures can lead to issues like excessive shrinking of the concrete, or the creation of zones with a weaker structure than the rest of the concrete. It's a balancing act to find the ideal conditions for speeding up hydration without creating these adverse conditions.
Predicting hydration rates through mathematical models relies heavily on accurately capturing how temperatures change within the concrete mass. If there are even minor inaccuracies in our thermal profiles, the prediction of the concrete's maturity – basically how far along it is in the hydration process – can be flawed, which can have big consequences for structural integrity.
Also, the concrete's ability to transfer heat, or thermal conductivity, is not constant. It depends on factors like the type of aggregate and the amount of moisture within the concrete, and these variations can make it hard to accurately predict how temperature will move through the material. This further complicates the effort to develop reliable hydration models.
While it is often observed that initially the concrete has higher compressive strength with elevated curing temperatures, the overall long-term durability can be negatively impacted if the temperature gradients are not appropriately managed throughout the curing process. The long-term performance of concrete, under a range of environmental conditions, is also sensitive to these factors. Ultimately, maintaining control of the temperature field during curing is essential for a wide variety of concrete applications to ensure concrete structures are able to perform as intended.
Temperature Impact on Water-Cured Concrete Analysis of Hydration Rates at Different Depths - Temperature Distribution Patterns Between Reinforcement Layers During Water Curing
When concrete is water-cured, the way heat is distributed between layers of reinforcing steel becomes very important for understanding how the concrete will behave. Higher temperatures can greatly influence how quickly water within the concrete evaporates, which can disrupt the hydration process and even weaken the structure's integrity. Because layers of reinforcement can essentially act like insulation within the concrete, differences in temperature between regions can become substantial. This uneven temperature distribution can create weak spots in the bond between the concrete and the reinforcement, which may lead to structural issues.
It's essential to carefully monitor and regulate temperatures within large concrete masses, especially in construction projects. Doing this can prevent things like thermal cracking, which is caused by uneven expansion and contraction due to temperature changes, and ensure that the hydration process progresses uniformly, leading to consistent strength across the entire concrete piece. Understanding these complex temperature patterns is vital for optimizing the curing process and ensuring that structures built with concrete are durable and dependable.
Examining the temperature distribution within the layers of concrete during water curing reveals a complex landscape of thermal gradients. The temperature differences between the surface and the core of a concrete element can be substantial, sometimes exceeding 30°C, highlighting a crucial challenge in achieving uniform curing conditions. This uneven distribution can significantly impact the hydration process, leading to variations in reaction rates across different layers.
Interestingly, while higher temperatures in certain layers may speed up the early stages of hydration, this doesn't necessarily translate to a more homogeneous final product. The complex interplay of heat transfer within the concrete can create a heterogeneous microstructure, where the arrangement of the constituent materials varies between zones. Factors like the type of aggregate used and the moisture content of the mix significantly affect the concrete's ability to conduct heat. These variations in thermal conductivity further complicate the already complex picture of temperature distribution, making accurate prediction challenging.
Another factor adding to the complexity is the phenomenon of self-heating. The hydration reactions themselves generate heat, creating localized hotspots within the concrete mass. This self-heating can significantly worsen the existing temperature gradients and contribute to the build-up of internal stresses, especially in larger concrete structures. Understanding how these hotspots develop and influence the overall temperature profile is essential for mitigating potential cracking risks.
Further complicating matters is the intrinsic temperature sensitivity of different cement types. Each blend responds differently to temperature fluctuations, with some requiring specific temperature ranges for optimal hydration. These sensitivities can directly influence the concrete's long-term mechanical performance, highlighting the importance of selecting cement types appropriate for the intended application and curing environment. Additionally, the volume of concrete plays a significant role in the development of these temperature gradients. Larger volumes can generate significantly higher internal temperatures, necessitating a scale-dependent approach to temperature management strategies.
The effect of pressure on hydration rates shouldn't be ignored either. As we move deeper into a concrete mass, pressure variations start to influence how the chemical reactions proceed. It's clear that sophisticated modeling approaches are needed to account for these depth-related pressure effects to gain a more complete understanding of how hydration develops within concrete.
The resulting variations in hydration lead to differences in the concrete's performance between the surface and the core. Surface curing techniques often lead to a dense microstructure, which can create a stark contrast to the potentially weaker and less-developed core. This disparity poses a risk, as the core might not achieve the same level of strength and stability as the surface, which could compromise the overall structural integrity.
The uneven temperature distribution caused by external temperature changes can also contribute to the development of thermal stresses. These stresses can potentially lead to macroscopic deformation or cracking if not properly managed, a significant concern for the long-term performance of concrete structures.
Current mathematical models used to predict hydration often simplify the complex interplay of temperature gradients within the concrete mass. This oversimplification can lead to inaccuracies in the prediction of concrete maturity and performance. To enhance the predictive power of these models, there's a clear need for ongoing validation against real-world data, continuously refining our understanding of the complexities of temperature distribution and its influence on concrete hydration. It's clear that accurately predicting the behavior of concrete during the curing phase necessitates continuous refinement of both our models and our understanding of these intricate temperature dynamics.
Temperature Impact on Water-Cured Concrete Analysis of Hydration Rates at Different Depths - Measuring Long Term Strength Development Across Different Temperature Zones
Understanding how concrete strength develops over time in different temperature environments is vital for predicting long-term performance. Studies suggest that while higher curing temperatures can accelerate initial strength development, they might not be ideal for long-term structural health, especially if temperature variations within the concrete mass are not properly controlled. The research reveals that hydration rates can differ substantially across varying depths, implying the potential for uneven strength and internal stress development. This can increase the risk of cracking, impacting the overall structural integrity. Finding a balance between speeding up the initial hydration and maintaining uniform strength across the entire concrete structure is crucial for durability. This knowledge is critical for making informed decisions during the design and construction phases, including choosing the right materials and implementing effective curing practices that promote optimal long-term concrete performance.
The pace of cement hydration is profoundly influenced by temperature, with a notable acceleration in the 15-60°C range compared to higher temperatures. However, the long-term implications of this temperature sensitivity on concrete strength remain an open question. While hot curing accelerates early strength development, it might hinder long-term performance, with 60°C appearing to be a pivotal point.
Continued hydration over long periods significantly impacts strength gains, with compressive strength potentially increasing by as much as 36% over several decades. This suggests that while initial strength development can be accelerated, the optimal curing temperature for long-term durability may be different. Studies indicate that higher curing temperatures lead to greater early strength, but it's unclear if this translates to superior long-term performance.
The observed strength variations appear to be primarily a consequence of modifications in the cement paste's chemical composition and the physical impacts of the curing environment. For example, a study examining cement-stabilized soil found that higher curing temperatures generally boosted strength over time. Similarly, hydration of alite in bioconcretes seems to improve with extended curing, demonstrating the importance of considering both the curing temperature and the duration of curing.
Extensive research has been undertaken to characterize the hydration and strength development of various cement types across a wide range of temperatures. One key observation is that the depth of a concrete pour significantly affects the rate of hydration, with differing temperatures leading to distinct hydration rates at different depths. This complex interaction of depth and temperature raises concerns about consistent strength development within the concrete. Furthermore, in massive concrete structures, variations in temperature can lead to thermal stresses, highlighting the importance of thermal stress analysis to predict and prevent cracking.
This dynamic interplay between temperature and the curing process makes managing hydration and promoting concrete strength a complex task. For instance, managing temperature fluctuations through the use of supplementary cementitious materials, like slag, is one way to control the rate of heat release and ultimately mitigate stress development. Accurate temperature prediction is critical for mitigating thermal cracking, particularly in situations with significant temperature changes. In essence, there are multiple factors influencing the outcome, making consistent control of temperature crucial. A deeper understanding of these relationships is vital for ensuring the long-term performance and durability of concrete structures.
It is important to consider how variations in curing temperature can potentially impact the final product, particularly the long-term performance of the material. While some studies suggest that higher temperatures may enhance short-term properties, there are risks associated with excessive temperature variations. This underscores the need for balanced approaches to optimizing temperature management in curing processes. In conclusion, while it is clear that temperature is a key driver of the hydration process, achieving consistent, durable concrete requires careful management and consideration of long-term consequences.
More Posts from aistructuralreview.com: